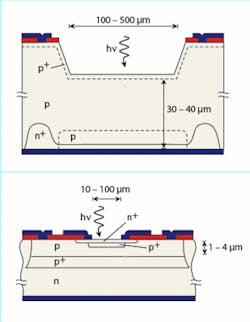
NICK BERTONE, ROBERTO BIASI, and BRUNO DION
Many applications in bioscience, imaging, and lidar require low-light detection. In fluorescence-correlation spectroscopy, for example, the confocal technique used to examine properties of single molecules requires single-photon detection (because of the minuscule amount of fluorescence generated from a single molecule). A novel detector technology based on avalanche photodiodes is well suited for single-photon detection in applications such as these.
Linear and Geiger modes
The simplest method of detecting light is to convert light energy into a voltage output using a detector-amplifier configuration. In such a configuration, the voltage output is directly proportional to the light intensity; here, the lowest light levels detectable occur when the signal-to-noise ratio (S/N) is unity, or when the output voltage equals the square root of the sum of the detector and amplifier noise. At this light intensity, the output from the amplifier equals the noise of the detector/amplifier combination. This value is referred to as the noise-equivalent power (NEP).
When the light being detected is continuous-wave (CW), cooling the detector decreases the detector noise and low-pass filtering can be used for minimizing the effect of the amplifier noise, thus achieving lower light detection. In many biomedical or lidar applications, however, the light to be detected is not CW, but is usually a short pulse with a fast decay. In this case, the amplifier noise is by far the dominant source of noise, whereas at low bandwidths its importance is strongly reduced. For example, a detector module with an NEP of 30 fW/√Hz can detect 20-ns pulses of light with a peak power of 0.2 nW. To detect lower levels, photon counting is required.
In linear-mode operation, the input power produces an output that is directly proportional to the input light intensity. In photon-counting mode—or Geiger mode, as it is often referred to—each photon that is detected generates an output pulse that is counted. It is very important to note that when photons are counted in this mode of operation, there is a so-called “dead time” before the next photon can be detected and counted; for example, if 106 photons arrive at the detector at the exact same time, only one pulse will be generated, indicating that only one photon was detected. If 106 photons were available at the same time for detection, however, then photon counting would not be required-linear-mode detectors would do just fine. If, on the other hand, only 25 photons per second were available for detection—which corresponds to an intensity of 0.01 fW—then photon counting would be required.
Single-photon avalanche diode
The solid-state detector used in this mode of operation is a single-photon avalanche diode (SPAD). A SPAD is a semiconductor-junction diode in which a self-sustaining avalanche-multiplication process can be triggered. Because SPADS are biased above the breakdown voltage (referred to as the overvoltage) they are are remarkably different from ordinary avalanche photodiodes (APDs), which work in linear amplifying mode biased slightly below the breakdown voltage.
At a defined overvoltage, the electric field within the depleted region of the device is very high, so that a single photon triggers a multiplication process in which an avalanche current of tens of milliamps flows through the junction. This current is then used to initiate a standard output pulse to indicate a photon has been detected, and the current rising edge signals the photon arrival time.
To detect the subsequent photon, the avalanche current must be quenched. This is accomplished by lowering the SPAD bias voltage below breakdown, then resetting it back to its overvoltage. Because the SPAD cannot detect any incoming photon during the time it takes to reset the SPAD to its overvoltage, it is desirable to have this dead time as short as possible. The simplest approach is passive quenching, which leads to very long dead times (200 ns or more); active quenching, which is commonly used, provides dead times shorter than 50 ns.
SPADs based on CMOS processing
Photon counting with specialized avalanche photodiodes has been commercially available for many years using SPAD devices with a reach-through structure (see Fig. 1). The advantages of this structure are the high photon-detection efficiency in the visible and near-IR region, and the large active diameter. The disadvantages are the high bias voltage (250 to 500 V) and the dedicated fabrication process. In addition, this process is not suitable for the production of monolithic arrays.
Recent progress has resulted in the development of commercial modules based on SPADs that are fabricated with planar structures processed using CMOS-compatible technology.1 These SPADs provide many advantages, such as ultrafast timing resolution, high fabrication yield and thus low cost, low power consumption for portable systems, and the possibility of producing monolithic arrays to create large-area photon-counting arrays. The main disadvantage of the planar SPAD is lower photon-detection efficiencies at longer wavelengths. The planar SPAD using CMOS-compatible technology has a thinner depletion layer (about 1 µm) than the reach-through APD; at longer wavelengths (near IR), this thin layer greatly reduces the detection efficiency. For shorter wavelengths (in the visible range), a reduced thickness of the depletion layer is sufficient to provide high efficiency; in fact, at wavelengths below 600 nm, the efficiency of the planar SPAD is even greater than that of the reach-through devices, because the neutral layer at the top surface is thinner and absorbs a smaller portion of the incident light.
Photon-detection efficiencies of greater than 55% can be achieved by applying an antireflection (AR) coating on the detector (see Fig. 2). The planar SPADs have fast timing resolution; recently, 35-ps timing resolution with a 100-µm detector at room temperature has been demonstrated.3 By comparison, modules using the reach-through SPAD are achieving timing resolutions in the order of 300 ps.Fast timing resolution for bioscience
Fluorescence-resonant energy transfer is used to follow proteins, enzymes, and other biological macromolecules as they twist, turn, and take new shapes. As these molecules undergo changes, changes occur in the fluorescence lifetimes because of the variations of spacing between the donor and acceptor (see Fig. 3).4 Using time-correlated photon counting with 50-ps timing resolution and a confocal setup to examine single molecules, researchers are able to measure conformational changes with angstrom resolutions.Sunney Xie at Harvard University (Cambridge, MA) has used this technique to study changes in an enzyme system. A frequency-doubled modelocked laser was focused down to a spot small enough to excite a single molecule, causing it to fluoresce. Each excitation pulse generated a fluorescent photon, which was detected with a photon-timing detector module. The photon delay with respect to the laser was measured and recorded. A sophisticated correlation analysis of the data made it possible to infer the conformational variations versus time. Because the distance variations were very small, timing resolution of a few tens of picoseconds was required from the photon-counting detector module. The module in this experiment used a planar SPAD, had ultralow dark-counting rate and timing resolution of better than 60 ps, and was developed by a research group led by Sergio Cova at Politecnico di Milano (Milan, Italy).
Lidar at 1064 nm
There are many applications for lidar, with the chosen laser wavelength based on what is being detected and ranged. In applications encompassing long distances, for example, 1064 nm is ideal, because high-power lasers at this wavelength are readily available. Detectors with high sensitivities are not readily available at this wavelength, however (see Fig. 4). Between 400 to 900 nm, photon-counting detectors with good photon detection efficiencies are available using CCDs, planar SPADs, and reach-through SPADs, but the sensitivities of all these detectors roll off dramatically at about 950 nm.A module has been developed that uses a hybrid photomultiplier tube manufactured by Intevac (Santa Clara, CA).6 The tube, which is referred to as an intensified photodiode (IPD), is sensitive from 900 to 1300 nm and has an active diameter of 1 mm. The IPD uses an active transferred electron photocathode and a gallium arsenide Schottky avalanche photodiode as an anode. The first-stage amplification consists of a very low-noise electron-hole multiplication resulting from energetic impact of photoelectrons on the top surface of the APD. This stage is then followed by traditional APD multiplication. These two processes are sufficient to overcome preamplifier noise and are therefore capable of photon counting.
Because this module operates in linear mode with high gain, both modes of operation-linear and Geiger-are possible. Modules have been produced with a 1-mm-active-diameter detector that have both photon-counting and analog outputs, photon-detection efficiencies of 30% at 1064 nm, dark counts of 3 × 104 counts per second, and an NEP of 9.4 × 10-15 W/√Hz when operating in linear mode.
REFERENCES
1. S. Cova et al., J. Mod. Optics 51, 1267 (2004).
2. F. Zappa et al., IEEE Photon. Technol. Lett. 17, 657 (2005).
3. A. Gulinatti et al., Electron. Lett. 41, in press (2005).
4. H. Yang et al., Science 302, 262 (2003).
5. I. Rech et al., IEEE J. Sel. Topics Quant. Electron. 10, 788 (2004).
6. Ross A. La Rue et al., IEEE Trans. Electron Devices 44 (4) (April 1997).
Nick Bertone is president of Optoelectronic Components, Box 122, Pierrefonds, Québec H9H4K8, Canada; e-mail: [email protected]. Roberto Biasi is chief executive officer of Micro-Photon-Devices, Via Stradivari 4 I-39100 Bolzano, Italy; e-mail: [email protected]. Bruno Dion is senior optoelectronic specialist at CMC Electronics, 600 Dr. Frederik Philips Blvd., Saint-Laurent, Québec H4M 2S9, Canada; e-mail: [email protected].