Raman Spectroscopy/Camera Technology: Melanoma detection with SWIR Raman spectroscopy
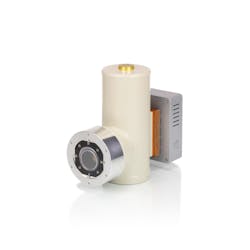
PETER J. CASPERS, PATRICK MERKEN, RAF VANDERSMISSEN, INES SANTOS, and GERWIN PUPPELS
"Raman spectroscopy is a very versatile technique to look at anything that you can shine light on," says Gerwin J. Puppels. Puppels is is founder of spectroscopy systems developer RiverD International B.V. (Rotterdam, The Netherlands) and one of six researchers at the Erasmus University Medical Center (Rotterdam, The Netherlands) who collaborated with RiverD International on an extensive project funded by the Netherlands Ministry of Economic Affairs to explore medical application of Raman spectroscopy.1 What makes the technique attractive for biomedicine is its power, sensitivity, and ability to deliver high-resolution quantitative analysis without destructive effect on—or even contact with—specimens.
Raman spectroscopy measures the inelastic scattering of light from the molecules of a specimen illuminated at low levels by monochromatic light from a laser or other source. The sample absorbs photons from the illuminating light source and re-emits them with a slightly shifted (up or down) frequency. The downshifted frequency, used in the application of the Raman effect, is called the Stokes frequency—its upwards-shifted counterpart is called "anti-Stokes." Stokes and anti-Stokes frequency parts represent a minuscule fraction of 0.001% of the total light reflected by the sample because of elastic scattering called Rayleigh scattering.
Separating the weak desired Stokes frequency part from the extremely energetic Rayleigh scattering requires an elaborate setup of apertures, filters, or multi-spectroscopic and tuning devices. To overcome these difficulties, various methods have been conceived and developed for sample illumination and detection. Among these methods are stimulated irradiation, coherent anti-Stokes or nonlinear stimulation, and surface-enhanced Raman spectroscopy (SERS) using signal emission from metallic surfaces. Thus, despite its inherent difficulties, researchers have successfully developed Raman spectroscopy for numerous applications.
The detector of choice for Raman spectroscopy has been a silicon-based CCD sensor. But the fact that usable responsivity range of a CCD tops out at 1 μm is a serious limitation for medical applications, especially for investigating and characterizing darkly pigmented tissue. In the visible spectrum, such specimens emit strong fluorescence, which severely impacts the obtained Raman spectra.
While researchers have made numerous attempts to mitigate analytical degradation by fluorescence—for example, with time-gated detection, photobleaching, confocal signal detection, SERS, and resonance Raman (RR) scattering—none have led to a suitable setup for in vivo Raman spectroscopy of pigmented tissues. Fourier-transform (FT) Raman spectroscopy, however, has demonstrated the ability to yield usable spectra of pigmented skin lesions.1 FT Raman proves that tissue fluorescence can be successfully sidetracked by deploying a longer laser excitation wavelength—for example, 1064 nm. For these reasons, the Erasmus researchers found FT Raman compelling, but they ultimately had to reject the technique. Because it is based on the multiplexing of single-channel analysis, FT Raman's signal integration times can exceed those of multichannel Raman spectroscopy by several orders of magnitude (it requires one to tens of minutes to investigate results in a single spectrum). "It provides nice scientific results, but is hardly usable in medical practice," Puppels says.
Raman in the SWIR
So, the team needed to take a different path. And there, they encountered another hurdle.
Thanks to the extremely weak intensity of the Raman signal, Raman spectroscopy's main limitation is noise floor—which is exaggerated when the detector adds readout noise. "If you observe a signal—say, 10,000 photons-the shot noise on the signal is 100 photons," explains Puppels. "This would give you a signal-to-noise ratio of 100. Unfortunately, the readout noise of the detector will typically add an additional several hundreds of electrons, and the Raman measurement is no longer shot noise-limited. That puts you in a very bad position—especially when encountering weak signals consisting of just 10,000 photons."
While CCD detectors typically add 2–3 electrons of noise, InGaAs cameras operable beyond 1 μm have traditionally delivered readout noise of up to several hundred electrons. The team needed a detector capable of reaching beyond 1 μm, but adding minimal noise.
They found their solution in a camera that had never been used for medical spectroscopy—a high-performance camera designed for extreme low-light-level imaging applications in the shortwave-infrared (SWIR) realm, and for such demanding applications as photoluminescence measurements in semiconductor manufacturing and failure analysis. Investigating the camera, the researchers found Raman spectroscopy listed among its applications. Puppels was intrigued. "This might be a very promising avenue opening up to collect the Raman spectrum in seconds instead of minutes and hours," he said.
The Xenics (Leuven, Belgium) Cougar-640 (see Fig. 1) incorporates an InGaAs focal plane array detector (XFPA-1.7-640-LN2) featuring 640 × 512 pixels (at a pixel pitch of 20 μm) and a 24-bit analog-to-digital converter (ADC). A source follower per detector (SFD) readout scheme enables ultra-low noise levels (to <20 e‐). Each pixel features a full-well capacity of about 480,000 electrons, and a conversion gain of 2.17 μV/e‐. Typical dark current is <20 e-/sec/pixel, at 77K sensor temperature and with a target emissivity of 5% and target temperature at 300K. Even lower dark current values are achievable with a liquid nitrogen (LN2)-cooled target (~77K).To demonstrate the feasibility of wavelength-shifted Raman spectroscopy, the team devised and built a complete SWIR multichannel instrument that included the camera (see Fig. 2). For a light source, they used a single-mode continuous-wave diode laser radiating at 976 nm—which provided output power of 150 mW (Model R-type from Innovative Photonic Solutions [Monmouth Junction, NJ]).Adjusting for medical applications
A key point of interest was the fact that the camera's readout scheme-called Read While Integrate (RWI)—enables lowering read noise by an order of magnitude. RWI, sometimes called "up-the-ramp readout," probes the accumulating photoelectrons through nondestructive sampling without resetting the buffering capacitors. The approach enables operation of the camera in extremely low-light-level applications, and it virtually eliminates effective readout noise.
Because the camera had never been used for medical spectroscopy, the team determined to enhance some of its characteristics by developing software to augment that supplied by Xenics. They wrote algorithms to read and pre-process the raw data that is delivered in the camera's RWI readout scheme.
Also, the camera's response curve showed a definite progressive nonlinear behavior when the accumulated signal exceeded a certain threshold, which necessitated a cutoff threshold for a more linear behavior. So, they devised an algorithm to correct the response above the threshold—for this purpose, they fitted a first-order polynomial to the linear range during the first part of the integration period. Figure 3 shows some tissue samples obtained with the experimental SWIR Raman spectroscopy setup.The RWI principle was devised for industrial applications such as inspecting semiconductor chips for leakage. Such measurement takes time while the signal builds up.
The Erasmus team's software operates similarly, nondestructively capturing the signal many times before producing a final averaged result. The effective noise level can be very low. The team reports having reduced from ~20 to ~2 e-, effectively.
The team found the detector's readout noise (in e-, with CDS) to be 22.7 e- (with 5.9 e- standard deviation), and dark current (e-/s/pixel) at 69.4 e-/s/pixel (with 4.5 e-/s/pixel standard deviation). Note that dark current strongly depends on target temperature and target emissivity. This noise level substantially decreases with more sampling readouts during the integration time. The final results given in the table indicate that the effective readout noise was reduced to the value comparable to cooled slow-scan CCD detectors.
The researchers caution that there are technical challenges yet to overcome. Raman spectroscopy's low signal-to-noise ratio implies limits for performing in the SWIR range to circumvent fluorescence effects produced by pigmented tissue in the visible realm. Not the least of these is cost. But an array with fewer pixels may be sufficient for medical applications, and such adjustments will be the focus of future work. In any case, this work is expected to substantially further the diagnosis of melanoma.
REFERENCE
1. I. P. Santos et al., J. Raman Spectrosc., doi:10.1002/jrs.4714 (2015).
Peter J. Caspers, Ph.D., is a researcher at Erasmus University Medical Center, Rotterdam, The Netherlands; Patrick Merken is CTO at Xenics, Leuven, Belgium; Raf Vandersmissen is CEO of sInfraRed Pte Ltd. - a Xenics company, Midview City, Singapore; Ines Santos is a PhD student at Erasmus University Medical Center; and Gerwin Puppels is founder of RiverD International, Rotterdam, The Netherlands; e-mail: [email protected]; www.erasmusmc.nl.