Photonic Frontiers: Extreme Fiber Bandwidth: The quest for extreme fiber bandwidth
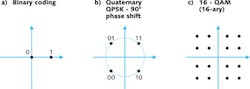
Over the past four decades, the fiber-optic bandwidth record has risen from tens of megabits per second to hundreds of terabits per second, roughly an order of magnitude every six years. The progress reflects several generations of technology, from multimode to single-mode to optical amplification and wavelength-division multiplexing (WDM) in the 1990s. The 2000s brought coherent transmission for 40 and 100 Gbit/s systems.
Now the quest for extreme bandwidth is pushing two more technological transformations. One now underway is a shift to sophisticated signal encoding that enhances fiber transmission capacity. One likely to follow is spatial-division multiplexing, with light signals taking parallel paths through a single fiber, either in separate modes or in separate fiber cores.
Coherent transmission and digital processing
Today's backbone networks are based on improved versions of the standard single-mode fiber introduced in the early 1980s. Installed cables represent a huge investment, so optical developers enhance capacity by using advanced multilevel coding techniques used in radio communications. Instead of traditional off-on binary coding of a single bit, they encode multiple bits per symbol by simultaneously modulating phase, polarization and/or amplitude, implementing techniques used in radio-frequency cellular networks. The result is a constellation of possible characters, as shown in Figure 1, which are resolved by coherent detection.1
Advanced modulation formats require coherent transmission to resolve more than one coding level per signal interval or symbol. Coherent systems operating at 100 Gbit/s line rates using WDM can send several terabits per second through standard single-mode fiber.2 The first 100 Gbit/s systems used polarization-division multiplexed quadrature phase shift keying (PDM-QPSK), a four-character constellation with a symbol rate of 28 Gbaud, which includes extra bits for forward error correction. The receiver includes an analog-to-digital converter and powerful digital signal processor to extract a digital signal. At 100 Gbit/s, says Peter Winzer of Alcatel-Lucent Bell Labs (Holmdel, NJ), "with 2X oversampling, you have an aggregate 200 Gsamples per second, each with 6 to 8 bits of resolution," producing a data bus of more than 1 Tbit/s at the processor to extract the 100 Gbit/s data stream.
The latest coherent systems use 16-ary quadrature-amplitude modulation (16-QAM). The receivers include digital-to-analog converters, so they can digitally generate analog pulses for high-speed modulation. That technology can be extended to 400 Gbit/s by ganging together two 16-QAM subcarriers. Field trials have reached line rates of 400 Gbit/s over a 350 km span in Australia and over a 400 km span in Canada. Line rates of 400 Gbit/s require wider WDM spacing than 100 Gbit/s, but can squeeze 17.6 Tbit/s into the C band over a few thousand kilometers.
Spectral efficiency can be increased somewhat by ganging together multiple signals at regularly spaced frequencies, such as combining five 200 Gbit/s signals to generate a 1 Tbit/s "superchannel." Eventually, says Winzer, you want to generate carrier frequencies collectively in a frequency comb rather than using separate laser sources. Because the comb lines share a common source, any drift is collective, making the superchannel more resilient to potential degradation at high speeds.
Shannon's efficiency limit
Laboratory tests of the most advanced coding techniques approach a fundamental limit on spectral efficiency, measured by the number of bits per second per Hertz of transmission spectrum. "We can pack 20 Tbit/s into the C band of erbium amplifiers, but we will not be able to reach 100 Tbit/s in the C band over thousands of kilometers," says Winzer.3
That theoretical limit was formulated in 1948 by information theory pioneer Claude Shannon, who found that it depends on the signal to noise ratio S/N. Modifying his formula by dividing data rate C (in bits/second) by bandwidth B (in Hertz) gives maximum spectral efficiency in bits/s/Hz:
Commercial systems spanning a few thousand kilometers are within a factor of 4 to 7 of the nonlinear Shannon limit.3 Hero experiments are within a factor of two. In a postdeadline paper at the European Conference on Optical Communications in September 2013, TE Subcom reported coming within 2.5 dB of the limit in a 9100 km experiment that reached 4.93 bits/s/Hz efficiency.5 Winzer says low-nonlinearity fibers "might be able to increase the limit by a factor of two or so, but not by the factor of 100 we are after."6
Spatial-division multiplexing
The Shannon limit applies to spectral efficiency on a single communications path. Total capacity can be increased by expanding bandwidth on that path, such as by transmitting in the 1565 to 1625 nm L band as well as the C band, but the spectral efficiency (in bits/s/Hz) remains limited. Avoiding the Shannon limit requires new communication paths-either parallel fibers, multiple cores in a fiber, or multiple modes in a fiber core, as shown in Figure 3. "The question is not if but when space-division multiplexing will be adopted in long-haul transmission systems," says Winzer.3Parallel fibers provide separate paths in current systems; some cables include hundreds of fibers. But developers want to integrate those optical paths within a single fiber to take advantage of the economies of integration.
Multicore fibers date back to a seven-core fiber reported in 1979 by S. Inao and colleagues at Furukawa Electric (Tokyo, Japan).7 The development of microstructured fibers revived the idea. It's now possible to make long lengths of multicore fiber, and to couple them to each other and to fiber amplifiers.
Two impressive hero experiments were reported at the 2013 ECOC. In one, a team from KDDI R&D Labs (Saitama, Japan) and Furukawa (Chiba, Japan) reported transmitting 140.7 Tbit/s through 7326 km of seven-core fiber, including seven-core, C-band erbium fiber amplifiers. Each core carried 100 Gbit/s on each of 201 WDM channels spaced 25 GHz apart. The group claimed a record for capacity-distance product—1.03 Ebit/s/km (1.03 × 1018 bits/s/km).8
In the second, a team led by Takayuki Kobayashi of NTT Laboratories (Yokosuka, Japan) reported transmitting a total of 688 Tbit/s—344 Tbit/s in each direction—through a 1500 km span of 12-core fiber using distributed Raman amplification. Six cores transmitted in each direction, with even channels transmitting on a 50 GHz grid and the odd channels transmitting at frequencies shifted by 12.5 GHz from the grid. The total bit rate-distance product was almost the same as in the KDDI/Furukawa experiment.9The same group had earlier reported a slightly higher data rate, but only through a 450 km span of 12-core fiber.10
Modal multiplexing is based on few-mode fibers, which support a handful of distinct spatial modes rather than the hundreds or thousands of modes in traditional multimode fibers. The modes are not as isolated as separate cores. "There is huge crosstalk among the modes," says Winzer, but tools developed for radio communications can cut the crosstalk. In the 1990s, mobile communications researchers found they could squeeze more signals through their limited slice of the radio spectrum by using multiple input and output antennas, an approach called MIMO.11 In a simple form, four antennas transmit different signals to an array of four receivers. The signals arrive scrambled, but digital signal processing can recover the four independent signals originally transmitted.
An optical version of MIMO allowed Winzer and colleagues at Bell Labs to achieve record spectral efficiency of 32 bits/s/Hz through 177 km of few-mode fiber, above the theoretical of 20 bit/s/Hz for a 200 km length of standard single-mode fiber. They transmitted 32 WDM channels spaced across an 800 GHz band on a total of 12 polarization and spatial modes (two polarizations in six spatial modes). Twelve receivers collected the signals, and digital processing decoded a total of 24.6 Tbit/s.12
Outlook for extreme fiber bandwidth
The quest for extreme bandwidth faces two big challenges. The first is developing economical and deployable technology for spatial division multiplexing in the backbone network. The second will be persuading carriers to invest in massive construction projects needed to install the new fiber—a problem that continues to stall fiber-to-the-home systems.
REFERENCES
1. P. J. Winzer, J. Lightwave Technol., 30, 3824 (Dec. 15, 2012).
2. J. Hecht, "High-speed fiber transmission reaches 100 Gbit/s on a single channel," Laser Focus World, 46, 3, 49–53 (Mar. 2010).
3. P. J. Winzer, "Spatial multiplexing: the next frontier in network capacity scaling," ECOC 2013, http://ieeexplore.ieee.org/xpls/abs_all.jsp?arnumber=6647590 (2013).
4. R.-J. Essiambre et al., "Capacity limits of optical fiber networks," J. Lightwave Technol. V, 28, 662–701 (Feb. 2010).
5. D. G. Foursa et al., "44.1 Tb/s transmission over 9,100 km using coded modulation based on 16QAM signals at 4.9 bits/s/Hz spectral efficiency," ECOC 2013, postdeadline paper PD3-E-1 (2013).
6. R.-J. Essiambre and R. W. Tkach, "Capacity Trends and Limits of Optical Communication Networks," Proc. IEEE, 100, 5, 1035 (2012).
7. S. Inao et al., "Multicore optical fiber," Optical Fiber Communications Conference, paper WB1 (Mar. 6–8, 1979); http://www.opticsinfobase.org/abstract.cfm?uri=OFC-1979-WB1.
8. K. Igarashi et al, "1.03-Exabit/s/km super-Nyquist-WDM transmission over 7,326-km seven-core fiber," ECOC 2013, postdeadline paper PD3-e-3 (2013); http://www.ecoc2013.org/docs/pd3-e-3.pdf.
9. T. Kobayashi et al., "2 × 344 Tb/s propagation-direction interleaved transmission over 1500-km MCF enhanced by multicarrier full electric-field digital back-propagation," ECOC 2013, postdeadline paper PD3-E-3 (2013); http://www.ecoc2013.org/docs/pd3-e-4.pdf.
10. A. Sano et al., Opt. Exp., 21, 14, 16777 (2013).
11. G. J. Foschini, "Layered space‐time architecture for wireless communication in a fading environment when using multi‐element antennas," Bell Labs Tech. J., 1, 2, 41–59 (1996).
12. R. Ryf et al., "32-bit/s/Hz spectral efficiency WDM transmission over 177-km few-mode fiber," OFC/NFOEC 2013, postdeadline paper PDP5A.1 (2013).
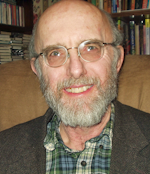
Jeff Hecht | Contributing Editor
Jeff Hecht is a regular contributing editor to Laser Focus World and has been covering the laser industry for 35 years. A prolific book author, Jeff's published works include “Understanding Fiber Optics,” “Understanding Lasers,” “The Laser Guidebook,” and “Beam Weapons: The Next Arms Race.” He also has written books on the histories of lasers and fiber optics, including “City of Light: The Story of Fiber Optics,” and “Beam: The Race to Make the Laser.” Find out more at jeffhecht.com.