Attosecond Technology: Visible-light pulses are only 380 attoseconds long
Scientists have for years been doing productive research using ultrafast light pulses with durations down to 2 or 3 fs. Now, researchers at the Max Planck Institute for Quantum Optics and Ludwig-Maximilians University (both in Garching, Germany), Texas A&M University (College Station, TX), and M.V. Lomonosov Moscow State University (Moscow, Russia) have pushed visible-light pulses beyond the femtosecond region into the attosecond (as) regime, creating pulses in the visible-light region only 380 as long.1 The group is headed by Eleftherios Goulielmakis, who is already well-known for his research into attosecond technology and science.
The new technique is in contrast to the more-usual production of attosecond pulses using high-harmonic generation (HHG), in which light is created over a very broad spectrum into the x-ray region, with the wide spectrum allowing the production of pulses under 100 as in duration (Goulielmakis and his lab are leaders in this area, too).
The optical attosecond pulses will enable not only science, as they for the first time provide direct access to the nonlinear response of bound electrons; they may also lead to photonic devices operating at subfemtosecond time scales and at petahertz (1015 Hz) rates.
Light-field synthesizer
The group created optical attosecond pulses using a light-field synthesizer that can manipulate the properties of visible light along with nearby infrared (IR) and ultraviolet (UV) frequencies—about 1.1 to 4.6 eV, or on the order of 1130 to 270 nm. The synthesizer managed broadband and almost dispersion-free spectral equalization of the synthesized pulses, producing pulses with twice the initial bandwidth. The resulting 380 as light pulses are so short that they are barely more than a half-oscillation of a light field in duration, making them the fastest pulses of visible light ever created.
First, broadband (1.1–4.6 eV) light pulses are generated via nonlinear broadening of laser pulses with 22 fs duration, 1 mJ energy, and 790 nm wavelength achieved by passing them through a hollow-core fiber filled with neon gas; the supercontinuum pulses have energies of about 550 μJ each.
Each of these pulses is then spectrally divided into four spectral bands: near-infrared (NIR; 1130–710 nm), visible (710–500 nm), visible-UV (500–350 nm), and deep-UV (350–270 nm). Each of the four resulting pulses is individually compressed via dispersive mirrors to durations of 8.5 fs for the NIR pulse, 7 fs for the visible, 6.5 fs for the visible-UV, and 6.5 fs for the deep-UV pulse. The four pulses are then recombined spatially and temporally to create the final attosecond pulse, which has an energy of about 320 μJ (see figure).
The physical overlaying of the four spectrally divided pulses to create the attosecond pulse must be done very precisely. In addition, the intensity of each of the four pulses is carefully controlled to achieve the proper spectral-band power in relation to the other three pulses. To produce longer, single-cycle pulses, the short-wavelength (<410 nm) end of the attosecond half-cycle optical pulse's spectrum can be optically removed-the resulting narrower spectrum leads to a longer pulse.
Resulting science
The researchers focused optical attosecond pulses onto a cell filled with krypton gas at a pressure of 80 mbar, generating vacuum-UV (VUV) spectra. A spectrometer placed downstream from the cell probed the resulting nonlinear polarization. The measured spectra ranged from 5 to 14 eV (250 to 89 nm)—this was possible because the sampled spectra do not overlap at all with the spectrum of the optical attosecond pulses. The results showed a finite nonlinear response time of bound electrons of up to 115 as, controllable via the field of the optical attosecond pulses.
The researchers say that it should be possible to extend the technique to the analysis of solids as well, with the potential to push the limits of optical signal processing by achieving ever-faster rates.
REFERENCE
1. M. Th. Hassan et al., Nature, doi:10.1038/nature16528 (Feb. 3, 2016).
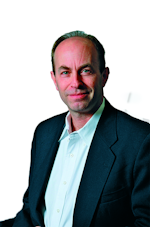
John Wallace | Senior Technical Editor (1998-2022)
John Wallace was with Laser Focus World for nearly 25 years, retiring in late June 2022. He obtained a bachelor's degree in mechanical engineering and physics at Rutgers University and a master's in optical engineering at the University of Rochester. Before becoming an editor, John worked as an engineer at RCA, Exxon, Eastman Kodak, and GCA Corporation.