3D-printed spectrometer could be integrated directly into distal end of endoscope
Since its introduction in 1806, endoscopy has revolutionized medicine by providing physicians a minimally invasive view inside the human body. Endoscopes have undergone several major advancements over the past 200 years, almost all of which have been tied directly to breakthroughs in optics and photonics. In a 2017 review titled “History of the Endoscope,” Piet De Groen, Professor of Medicine at the University of Minnesota, provided a detailed timeline connecting endoscopy breakthroughs to events such as the invention of the light bulb, the wide-angle lens, fiber optics, and CCDs.1
While this review is exceptionally comprehensive, it does overlook one of the more exciting developments in endoscopy: spectroscopic endoscopes. The integration of spectral probes in endoscopes provides clinicians with the ability to perform biochemical analysis in real time without the need to remove any tissue.
The pairing of spectroscopy and endoscopy is not a new idea: publications on the topic can be found dating back as far as 1990.2 In one of the earliest papers on the subject, teams from the Cleveland Clinic Foundation and the Massachusetts Institute of Technology reported the development of a fiber-optic endoscope to “accurately diagnose mucosal abnormalities” using fluorescence spectroscopy. At the time of this initial work in the field, photonics technology had not yet matured to the point where it was feasible to deploy spectroscopic endoscopes commercially.
In a 2003 book on Barrett’s esophagus by Guido Tytgat, President of the World Gastroenterology Organization and Professor at the University of Amsterdam, he stated, “Clinical application of [endoscopic] fluorescence detection of dysplasia and early cancer is still in its infancy.”3 Unbeknownst to Dr. Tygat, around the time of that publication spectroscopic instrumentation was entering into a period of rapid advancement, fueled by the subsequent telecom boom-and-bust at the turn of the century. Since then, spectrometers and light sources have become smaller, less expensive, and easier to use, which removes many barriers to spectroscopic endoscope clinical deployment.
Trends in endoscopic spectroscopy probes
Fiber-optic endoscopes were initially introduced in the 1950s to produce flexible light pipes for imaging purposes. The easiest way to visualize this is to envision a bundle of fiber-optic cables, such as a CCD array, where the individual fibers in the bundle are analogous to individual pixels on the CCD. In practice, one or more large-core fibers were typically placed in the bundle’s center to be used as an illumination source, making the analogy more akin to a CCD with a few dead pixels. As camera technologies continued to miniaturize, it became possible to directly incorporate the camera into the distal end of the endoscope. This greatly reduced the number of fiber optics needed and increased the scope’s flexibility.
This also meant that the existing fiber optics would then be repurposed to allow for various spectroscopies. Figure 1a shows a schematic example of the distal end of a typical spectroscopic endoscope. While exact configurations can vary widely, the basic principle tends to remain the same: the camera and illuminator are recessed slightly from the spectral probe tip. This configuration allows the clinician to obtain visual confirmation that the probe tip is in contact with the specific target region. To date, endoscopic spectral probes using Fourier transform infrared (FTIR) spectroscopy,4 Raman spectroscopy,5 fluorescence spectroscopy,6 and multiphoton spectroscopy7 have all been shown to be effective in diagnosing cancers and other diseases.
FTIR spectral probes utilize attenuated total internal reflectance (ATR) to detect infrared (IR) absorption when in contact with tissue (see Fig. 1b). Typically, Raman probes use a ball lens to focus the excitation laser at the contact point while simultaneously collecting the scattered light into a collection fiber (see Fig. 1c). While not consistently implemented, it is generally considered best practice to place the laser line (short-pass) filter and Rayleigh (long-pass) filters at the fiber’s distal ends. Otherwise, there is a high likelihood that the laser will generate a significant Raman signature inside the fiber-optic cable, which can interfere with the signal from the tissue under investigation.
Due to the simplified nature of the technique, fluorescence probes can be constructed far simpler. They typically consist of a central excitation fiber surrounded by a bundle of collection fibers (see Fig. 1d). A hollow ring filter may be used in a fluorescence probe to block the excitation light from entering the collection fibers, or this can also be implemented at the proximal end if preferred.
The future of endoscopic spectroscopy probes
Today’s spectroscopic endoscopes are making remarkable strides in medical diagnostics—however, they suffer from one major drawback: bend radius. Typical fiber-optic cables have a minimum bend radius between 10 and 20X the outer diameter. By contrast, typical copper wire cables have a minimum bend radius as low as 4–5X the outer diameter. As a result, fiber-based endoscopes are limited in their ability to fit into narrow winding musculatures such as Fallopian tubes and intestines.
A group from the University of Stuttgart recently took minimization to a whole new level when producing ultra-miniature 3D-printed spectrometers with a less than 100 × 100 × 300 μm3 total footprint.8 This truly groundbreaking work, which was featured as a Newsbreak in the April 2021 issue of Laser Focus World, may now make it possible to integrate the spectrometer itself directly into the distal end of the endoscope. This would eliminate the need for collection fibers, dramatically reduce the overall diameter of spectroscopic endoscopes, and decrease the minimum bend radius.
The group in Stuttgart, led by Professors Herkommer and Giessen, produced the highly complex submicron freeform optical features using a novel 3D-printing technology known as direct laser writing (DLW). This method uses two-photon absorption to induce highly localized nonthermal polymerization in the precursor, which is limited only by the laser beam’s focal volume. For this application, the team produced features as small as 650 nm using a femtosecond fiber laser centered at 780 nm.
The laser was scanned at a rate of 15 mm/s, leading to a total print time of roughly 2 hours per spectrometer. After the main optical features were printed, a super-fine inkjet printer provided the necessary baffling and light blockage for the entrance slit. This process resulted in the fully monolithic ultra-miniature spectrograph (see Fig. 2).Taking advantage of the 650 nm resolution of the 3D printer, the researchers managed to produce a chirped grating with a groove period ranging from 650 nm to 860 nm. This grating was etched directly into the lower optical element’s curved surface, allowing it to function as both the diffractive and focusing optic simultaneously.
Because of the high groove density, the spectrograph managed to produce an unprecedented 200 nm spectral range over a region of approximately 30 μm (see Fig. 3), with a spatial-spectra resolution of 9.2 ± 1.1 nm/μm at 532 nm. In the paper published in the open-source journal Light: Advanced Manufacturing, Andrea Toulouse et al. commented on the fact that even though their spectrometer would require a detector with sub-micron pixels, “[c]urrent trends in this direction are evident.” They went on to explain that “[f]or example, Samsung has announced a 47.3 MP sensor with a 0.7 μm pixel pitch (ISOCELL SlimGH1).”It is important to note that while ultra-miniature 3D-printed spectrometers may replace fiber-optic spectral probes in some applications, there are still fundamental tradeoffs between spectrograph size and resolution. As a result, it is doubtful that these types of spectrometers will ever provide the resolution required for high specificity spectroscopies such as Raman and FTIR. Still, many other spectroscopic techniques require far courser resolutions for which these devices may be ideally suited.
For example, near-infrared (near-IR) spectroscopy would be a perfect choice for a spectroscopic probe with an ultra-miniature 3D-printed spectrometer because of the inherent broad spectral features in that region. Toulouse et al. specifically noted in their paper that the photoresist used to construct these devices had an extremely low absorption coefficient in the IR region, making it ideal for near-IR spectroscopy applications. Laser-induced fluorescence spectroscopy is another example of a widely used spectral endoscopy technique, requiring a relatively coarse spectral resolution.
Looking to the future, it is abundantly clear that real-time spectral analysis will continue to be an active area of biomedical diagnostics. And endoscopic spectral probes, both fiber-optic and potentially directly embedded spectra sensors, are likely to become one of the most critical components in a surgeon’s toolkit over the next 5–10 years.
REFERENCES
1. P. C. De Groen, Proc. IEEE, 105, 10, 1987–1995 (2017).
2. R. M. Cothren et al., Gastrointest. Endosc., 36, 2, 105–111 (1990).
3. R. Giuli (ed.), Barrett’s Esophagus: Columnar Lined Esophagus: 250 Questions, 250 Answers (Vol. 1). John Libbey Eurotext (2003).
4. M. A. Mackanos and C. H. Contag, Trends Biotechnol., 28, 6, 317–323 (2010).
5. E. Cordero, I. Latka, C. Matthäus, I. W. Schie, and J. Popp, J. Biomed. Opt., 23, 7, 071210 (2018).
6. S. A. Goryaynov et al., Front. Oncol., 9, 830 (2019).
7. F. Akhoundi, Y. Qin, N. Peyghambarian, J. K. Barton, and K. Kieu, Biomed. Opt. Express, 9, 5, 2326–2335 (2018).
8. A. Toulouse, J. Drozella, S. Thiele, H. Giessen, and A. Herkommer, Light: Adv. Manuf., 2, 1, 1–11 (2021).
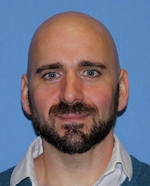
Robert V. Chimenti | Director, RVC Photonics LLC
Robert V. Chimenti is the Director of RVC Photonics LLC (Pitman, NJ), as well as a Visiting Assistant Professor in the Department of Physics and Astronomy at Rowan University (Glassboro, NJ). He has earned undergraduate degrees in physics, photonics, and business administration, as well as an M.S. in Electro-Optics from the University of Dayton. Over a nearly 20-year career in optics and photonics, he has primarily focused on the development of new laser and spectroscopy applications, with a heavy emphasis on vibrational spectroscopy. He is also very heavily involved in the Federation of Analytical Chemistry and Spectroscopy Societies (FACSS), where he has served for several years as the Workshops Chair for the annual SciX conference and will be taking over as General Chair for the 2021 SciX conference.