Single-photon detection has become a vital tool in many applications, from single-molecule fluorescence, particle characterization through scattering, and quantum cryptography to astronomy, lidar, and more. The concept appears to be simple in principle—one photon creates one electron in a photodiode or when hitting a metal surface—but how easy is it to detect that single electron?
Development of single-photon counting stems from discovery of the photoelectric effect in the late 19th century. Later, it was achieved in silicon avalanche photodiodes (APDs) in the 1960s. Today, turnkey modules provide simple, plug-and-run photon detection in OEM instruments and research laboratories alike. Single-photon detection is now enabling quantum communications, as it’s being deployed in space and underground in fiber networks, and in new single-molecule and small-particle characterization applications, with future development and uses continuing to emerge.
Early scientific discoveries
Late in the 19th century, Heinrich Hertz’s 1887 observations sparked experiments investigating the effect of light, particularly UV radiation, on charged bodies. Scientists then discovered that while the number of particles (electrons) emitted from a surface was proportional to light intensity, the electron’s maximum kinetic energy was proportional to radiation frequency, and that no electrons are emitted below a minimum frequency (see Fig. 1).
This work, coupled with Max Planck’s studies on blackbody radiation, led to Einstein’s Nobel Prize-winning paper proposing that light energy is carried in discrete packets (photons); the energy within each packet equals the light frequency multiplied by a constant (explaining the photoelectric effect); and only photons with high-enough frequency have enough energy to liberate an electron from a particular material. Since a photon can release an electron from a metal surface, a single photon can be detected by finding the negatively charged electron. Although simple in concept and relatively easy to do when large numbers of photons liberate large numbers of electrons, detecting one single electron is complicated.
Technology development
In 1902, Austin and Starke discovered that metal surfaces impacted by electron beams in a cathode-ray tube emitted more electrons than were incident onto the surface, leading to application of secondary emission to amplify signals. In 1934, RCA created the first photomultiplier tube (PMT) by combining a photocathode (creating electrons through the photoelectric effect) and a secondary emission stage in the same vacuum tube (see Fig. 2).With an approximate gain of 8, the PMT enabled the single photon to liberate eight electrons to prove its presence. With further improvements in photocathodes and multiple amplification stages, a typical gain of 106 is now possible in linear mode.
However, for single-photon detection, voltages across multiplication stages can be increased to raise the gain so high that a single photoelectron from the photocathode produces a very large current at the output circuit. This process tends to be self-sustaining, so control electronics are needed to detect the current and reset the PMT, leading to dead time where no electrons can be multiplied and therefore no photons detected. This method of operation, similar in principle to that of a Geiger counter, is known as Geiger mode.
Since the photocathode is far from 100% efficient, not every photon will generate an electron. Different photocathode materials respond to different wavelength ranges and have different photon energies below which they will not emit electrons. Electrons can also be emitted by other means (e.g., thermionic emission, where thermal energy boosts the electron enough to escape the electrode), giving rise to “dark electrons,” which, when operated in Geiger mode, leads to “dark counts” with no photons present.
Because the PMT can detect low levels of light, even down to the single photon, it has become an important tool in many applications including astronomy, nuclear particle physics, and biomedical instrumentation. However, PMTs are very sensitive to overstimulation and are easily damaged by exposure to ambient light. They operate typically at a 1–2000 V difference between the anode and cathode, with the anode at low voltage to allow for easier measurement of the photocurrent by low-voltage circuitry—so the cathode is at a large negative voltage. The PMT is also susceptible to magnetic fields, which can cause electron paths to curve and miss their targets, reducing gain; therefore, magnetic shielding is needed often at the cathode potential, creating a need for additional electrical insulation.
When Bell Laboratories invented the p-n junction in 1939, another route to single-photon detection—the photodiode—emerged. The p-n junction is the interface between differently doped regions within a single crystal of semiconductor. The p (positive side of the junction) lacks electrons where they would be expected within the crystal lattice; the missing electrons are termed “holes.” The n (negative) has an excess of electrons in the outer shells of the otherwise neutral atoms in the crystal lattice. This creates a diode, allowing passage of electrical current in only one direction under normal operation. A photodiode in its simplest form is a p-n junction whose materials enable the right radiation frequency or wavelength to release electrons and create a photocurrent within the junction. When reverse-biased with the cathode voltage raised positive compared to the anode, this photocurrent can be quickly extracted to produce a current proportional to the light level on the photodiode.
Adding an undoped region (“intrinsic” semiconductor) between the p- and n-type regions allows doping levels to be increased, causing higher levels of charge carriers and therefore greater operation speed. A PIN junction, invented by Jun-ichi Nishizawa et al. in 1950, is also ideal for a photodiode. Routine applications using PIN photodiodes range from fiber-optic communications to medical instrumentation and laser warning systems. But they are still limited to one photon creating one electron.
Avalanche photodiodes
Adding an internal current gain region within a PIN photodiode turns it into an APD (see Fig. 3). Invented by Nishizawa in 1952, the APD uses a careful doping structure to allow high-voltage application, creating high fields within the junction region.These high fields accelerate the photoelectrons, causing them to release other electrons through impact ionization and create a typical internal current gain of 100. Thus, a single photon can create 100 photoelectrons—however, this is still not enough to enable a simple single-photon detector.
In the 1960s, Robert McIntyre at RCA Canada (part of the same organization that developed the PMT, where Excelitas now makes its single-photon counting modules [SPCMs]) researched microplasma instability in silicon. This, in turn, led him to research APD behavior in Geiger mode, where the high reverse-bias causes the APD’s dark current to spontaneously create a self-sustaining avalanche. With an APD in Geiger mode, a single photon can produce enough current to be detectable. However, once a single photon has created the avalanche and a current is generated, the APD becomes useless without a method to control it. After the single photon has been detected, nothing else ever will be; this is not particularly useful.
Getting closer
RCA’s work led to two key developments in the search for a working single-photon detector. First, improvements in silicon crystal growth led to the 1986 super-low ionization coefficient (k-factor) APD, or SLiK (see Fig. 4). Since it has no photo- and negligible dark-electron stimulus, the SLiK APD can be biased to above breakdown without immediately initiating an avalanche.Cooling the APD can significantly reduce the thermionic emission of electrons, increasing the time available to detect an incoming photon.
To stop the avalanche, and allow the detection of another photon, an in-line resistor to the bias circuitry is added, creating a passive quench circuit (see Fig. 5). When the current starts flowing, some of the bias voltage is dropped across the resistor, leaving less voltage across the APD until it becomes so low that statistical variations in the photocurrent cause it to drop to zero and it cannot self-start. This causes the voltage across the resistor to return to zero, leaving the full voltage across the APD, which is now ready to detect another photon.Launched by RCA in 1987, the SPCM-100 was a self-contained, user-friendly device with built-in temperature control, stabilized high-voltage supply, and a Geiger-mode APD passive-quenching circuit. An on-board logic circuit detected the avalanche current pulse and generated a simple TTL pulse of 35 ns, and the passive quench circuit readied the APD for the next photon after only 60 ns. With its low dark-count, low timing jitter, low after-pulse, and a photon-detection efficiency (PDE) of over 50%, this first-generation module enabled single-photon studies to move deeper into red and near-infrared regions of the electromagnetic spectrum that were difficult to reach with PMTs. APDs’ much lower bias voltages, and their immunity to magnetic fields, eliminates the need for complex shielding, and APDs are also less vulnerable to accidental ambient light exposure. This development made single-photon detection readily achievable, becoming a useful tool to enable new discoveries and applications.
In 1990, RCA Canada became EG&G, and SPCM development continued. Passive quenching was replaced by a patented active-quench circuit, using the logic that detected the avalanche current to actively control the bias across the APD, rather than relying on voltage build-up across a resistor. Pulse width and dead time were reduced, and better control of bias voltage and silicon purity enabled increased PDE to >65% and decreased dark-count rates to 25 counts per second.
EG&G acquired the Analytical Instrumentation Division of PerkinElmer in 1999, upgraded the SPCM with a pulse width of 20 ns and dead time of 35 ns, and established its RoHS compliance. In 2010, the optoelectronics division of PerkinElmer was spun out to create Excelitas Technologies. In 2011, improved control electronics allowed PDEs as high as 70% while retaining industry-leading specifications for dark count and after-pulsing. The pulse width and dead time were both further reduced, affording 10 ns and 24 ns, respectively, to reliably detect over 37 million single-photon events per second.
Expanding applications
The market and applications for single-photon detection continue to expand. Different APD technologies for detecting single photons optimize key performance parameters to suit different applications. For automotive lidar at 905 nm, where cost is important for volume production, single-photon silicon avalanche detectors use micro-APDs on top of a CMOS structure. New indium gallium arsenide (InGaAs) Geiger-mode APDs can detect single photons at 1.5 µm, with higher-power lasers allowing significantly greater range to detect photons that are reflected back by objects around the vehicle.
InGaAs APDs for single-photon detection will also play a vital role in infrastructure and banking security as quantum key distribution (QKD) systems are deployed on fiber-optic links at telecom wavelengths. QKD allows two parties to set up a secure cryptographic key remotely in real time, without needing physical contact to share the key. QKD has already been demonstrated in free-space links on the ground and in satellite-ground and satellite-satellite links, using both silicon and InGaAs APDs.
As bandwidths increase, not only is photon detection important, but jitter in the time delay from the photon reaching the surface of the APD and the system output pulse being registered also becomes a key performance parameter. QKD relies on comparing a sequence of photons arriving at various detectors—if the internal delay time varies too much, it will be impossible to know which photons are being compared. APDs with small surface areas and thin structures can create the avalanche with much less variability in time delay, so their reduced detection performance compared to larger APDs is tolerated for applications where timing resolution is important.
Many applications leveraging the quantum nature of single atoms or electrons also need to be able to detect single photons. A single electron trapped in a crystal defect can only emit one photon at a time, so a single-photon detector is needed to understand this electron’s interactions. Creating an entangled photon pair starts with a single photon—again, the single-photon detector is a vital tool for characterization and monitoring of single-photon sources.
With development of ever-smaller features on electronic wafers, air and water cleanliness in semiconductor wafer fabs becomes more critical to their operation. Single-photon counters with high detection efficiency and low dark-counts are often key parts of monitoring systems in ultra-clean workspaces. High detection efficiency minimizes estimations for translating detector response to contamination levels, which reduces the likelihood of missing small increases in contamination levels. On the other hand, a low, stable dark count reduces false counts that may lead to false alarms and cause unnecessary and expensive shutdowns.
Recently, detection of small particles and single molecules has become an important application for single-photon detection. This technique provides ultimate sensitivity for environmental monitoring or diagnostic measurements.
SPCMs continue to support applications including astronomy, flow cytometry, fluorescence lifetime, particle sizing, and wind lidar, as well as recent and developing applications including but not limited to quantum computing, QKD, and single-molecule analysis.
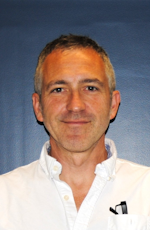
Richard Simons | Product & Applications Manager, Low-Light-Level Detection at Excelitas Technologies
Richard Simons is the Product Manager for Low-Light-Level Detection at Excelitas Technologies, based in their Montreal, QC, Canada site. He has been with the company for 10 years, as a sales manager and then Senior Application Specialist prior to his current role. He has an extensive background in photonics, with experience in high-power lasers, high-energy laser optics, fiber optics for telecommunications and sensors, as well as lidar and single-photon detection.