When it comes to detectors, single-photon detectors (SPDs) are the workhorse of photonics research. Single-photon detectors such as avalanche photodiodes (APDs) have evolved over the past two decades and are still among the most sensitive detectors that exist in photonics. The ability to detect a single photon with >90% efficiency played a vital role in the experiments confirming quantum entanglement, for which three researchers won the 2022 Nobel Prize in physics in October: Alain Aspect, John F. Clauser, and Anton Zeilinger.1 Their work with these small sensors confirmed quantum entanglement of two particles and demonstrated quantum teleportation, enabling a new age of emerging quantum technology.
Commercially available near-infrared (near-IR) and visible wavelength SPDs are efficient and especially useful in applications like quantum key distribution (QKD) and LiDAR. Cutting-edge development in SPDs is now focused on expanding into the mid-infrared (mid-IR) wavelength range, which has advantages in quantum sensing, as well as in applications involving rotational-vibrational molecular excitation, such as in exoplanet studies, gas molecule detection, fluorescence imaging, and spectroscopy.2 The challenges to mid-IR detectors involve lower detection efficiencies, higher timing jitter, and higher dark count rates compared to those in the near-IR.
One of the most promising recent mid-IR detectors in development is a superconducting nanowire single-photon avalanche diode (SNSPD), essentially a p-n junction that operates in Geiger mode—slightly above the breakdown threshold voltage for which a single electron-hole pair triggers a strong avalanche. SNSPDs have low dark count rates, improved detection efficiencies, and lower jitter compared to older types of mid-IR detectors. In addition, a single photon can easily and efficiently travel along the nanowire channel of an SNSPD, which is an essential element in secure QKD communications.
Researchers at the University of Glasgow (Glasgow, Scotland) demonstrated the high efficiency and timing performance of SNSPDs by applying them to a study of QKD at 2.1 µm in the mid-IR region of the spectrum.3 Assistant professor of optics Adetunmise Dada and colleagues were the first to implement device-independent QKD using niobium titanium nitride (NbTiN) thin-film SNSPDs, achieving near-maximal two-photon entanglement with a secure key rate of 0.254 bits/pair and a quantum bit error rate of 3.8%. Observing quantum efficiencies of up to 100% from the detector in the range from 2 to 3 µm, the group believes use of SNSPDs in the mid-IR is a promising solution for daytime free-space quantum communications, as well as ultrasensitive LiDAR systems.
Detectors developed for quantum applications may also someday help solve other fundamental problems in physics, such as gravity waves, neutrinos, and dark matter, according to Or Katz, a researcher in quantum physics at Duke University (Durham, N.C.).
“We believe that the technology developed for precision measurement or quantum sensing can be applied to detect and test new physics beyond the Standard Model of particle physics,” says Katz.
The Standard Model is the current theory that best explains three of the four fundamental forces that make up the universe: electromagnetism, the strong nuclear force, and the weak nuclear force. But the Standard Model does not adequately explain the fourth force, gravity, nor its place in particle physics, which is the purpose of pursuing a Grand Unified Theory. If the universe contains gravity particles, we have yet to detect them.
In pursuit of dark matter
Scientists also have yet to directly detect dark matter, the mysterious force that causes gravitational lensing around distant stars and galaxies but doesn’t appear to interact with light or ordinary matter. Numerous ways exist to study these mysteries.
For example, researchers at Tel-Aviv University, The Hebrew University in Jerusalem, and the Weizmann Institute of Science (all in Israel) are searching for nongravitational interactions of dark matter with regular matter.
Katz (previously with the Israeli groups, now at Duke University) and colleagues conducted a five-month-long search, the first of its kind, called the Noble and Alkali Spin Detectors for Ultralight Coherent darK matter (NASDUCK) collaboration.4 They used a Floquet quantum detector to observe spin-polarized particle interactions of xenon-129 (129Xe) gas with background dark matter as the Earth travels through the galaxy. A Floquet detector is comprised of a glass enclosure containing dense, spin-polarized 129Xe and rubidium-85 (85Rb) gas, pumped and probed by distributed Bragg reflector (DBR) lasers at 795 nm, and subject to an applied electromagnetic Floquet field (see Fig. 1).
NASDUCK’s goal is to detect hypothetical elementary particles called axions, or axion-like particles, that might interact with the spin of the xenon particles. Axions may be able to resolve the “strong charge conjugation and parity” problem in quantum chromodynamics, and could possibly be a component of dark matter. Their study did not detect axions or dark matter, but enabled constraints on the upper mass limit of axion-like particle interactions with neutrons, to between 4 × 10-15 and 4 × 10-12 eV/c2. The newly derived limits are a substantial improvement to previous bounds, and will help frame future searches.
“The advance of laser technologies to control and probe atoms and molecules, whose properties are very precise, is a prime technology for novel future detectors,” says Katz.
Another approach to detecting ultralow-energy phenomena is to go large. To detect gravity waves and neutrinos, scientists are placing sensors far apart on a planetary-scale, hundreds or even thousands of miles apart. For example, neutrinos are common subatomic neutral particles, nearly massless, that form in nuclear reactions deep in the heart of stars. They hurtle through space at near light speed, and when they reach a mass like the Earth, they pass right through without interacting. Billions of neutrinos pass through our bodies every second. We have many questions about neutrinos: Do they contribute to dark matter? What is their mass? How much energy do they have? But they are very difficult to detect.
Neutrino detectors
Enter the Deep Underground Neutrino Experiment (DUNE), a groundbreaking, multinational effort to build a series of long-baseline neutrino detectors, the first of which are being built now for planned operation in the mid 2020s. The first detector at the Fermi National Accelerator Lab in Batavia, Illinois, will monitor neutrinos produced by its own beam (via particle accelerators) while the second detector, a massive underground tank of liquid argon supercooled to -300°F, will reside underground 800 miles (1300 km) away at Sanford Underground Research Facility in Lead, South Dakota (see Fig. 2). The underground journey of the neutrino beam through the Earth will cause the particles to oscillate, and possibly change “flavors.” Some neutrinos will interact with the argon, leaving charged current ion and photon “trails.” The Sanford detector may also capture neutrinos from natural sources such as supernovae.Two other detectors will reside in Europe, even farther from the source at Fermilab. The vast majority of commercially available detectors are unable to detect the ultraviolet (128 nm) photons emitted when neutrinos excite the argon, so scientists are developing several detector designs.
Light traps
One type of design, being built by researchers at Indiana University, Fermilab, and MIT, are guiding bars, which capture light and guide it to an external sensor, much like fiber-optic cables. Counterparts in Brazil have developed a “light trap” detector configuration called Arapuca, an indigenous Brazilian word for “bird trap.” One of the latest versions of a single Arapuca trap is a centimeter-scale reflective box that allows a 128 nm photon in, trapping it and “downshifting” its energy within several layers: internal reflective coatings, a dichroic cutoff filter, liquid argon layers, and a wavelength shifting guide.5 The photon is then directed to silicon photomultipliers, where it’s neatly detected at 440 nm. Thousands of guiding bars and/or Arapuca arrays will be installed in the prototype phase DUNEs now being built, and eventually in the actual DUNE facilities.
The Arapuca light trap is an evolving experiment, being continually improved as the DUNE program takes shape. How well it will detect and measure neutrinos remains to be seen and leaves us hopeful for answers to some very important questions in physics.
REFERENCES
1. A. Aspect, Physics, 8, 123 (2015).
2. S. Dello Russo et al., Photonics, 9, 470 (2022).
3. A. C. Data et al., Phys. Rev. Appl., 16, L051005 (2021).
4. I. Bloch et al., Sci. Adv., 8, eabl8919 (2022).
5. E. Segreto et al, JINST, 13, P08021 (2018).
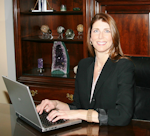
Valerie Coffey-Rosich | Contributing Editor
Valerie Coffey-Rosich is a freelance science and technology writer and editor and a contributing editor for Laser Focus World; she previously served as an Associate Technical Editor (2000-2003) and a Senior Technical Editor (2007-2008) for Laser Focus World.
Valerie holds a BS in physics from the University of Nevada, Reno, and an MA in astronomy from Boston University. She specializes in editing and writing about optics, photonics, astronomy, and physics in academic, reference, and business-to-business publications. In addition to Laser Focus World, her work has appeared online and in print for clients such as the American Institute of Physics, American Heritage Dictionary, BioPhotonics, Encyclopedia Britannica, EuroPhotonics, the Optical Society of America, Photonics Focus, Photonics Spectra, Sky & Telescope, and many others. She is based in Palm Springs, California.