ULTRAFAST LASERS: Free electron lasers thrive from synergy with ultrafast laser systems
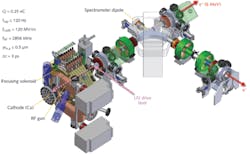
New generations of free electron lasers (FELs) provide high-brightness femtosecond pulses of coherent x-rays at wavelengths shorter than 0.15 nm, and with peak powers up to 20 GW. Picosecond and femtosecond Ti:sapphire amplifiers are used to generate the initial electron bunch, to optimize the FEL beam characteristics, and to perform time-resolved experiments in conjunction with the x-ray beam lines.
In a free electron laser (FEL), a beam of relativistic electrons passes through an undulator, a series of periodically spaced magnet assemblies with alternating polarity. The magnetic fields cause the electrons to accelerate in a sinusoidal path, resulting in the emission of electromagnetic radiation. The emitted radiation modulates the energy and density of the electrons to form "microbunches" that are spaced at the wavelength of the radiation. The microbunched beam then radiates coherently at the wavelength of the radiation.
Conventional x-ray sources, such as synchrotrons and x-ray tubes, produce incoherent x-ray pulses whereas FELs emit a stimulated, coherent output beam with much higher brightness and shorter pulse durations. The FEL emission wavelength depends essentially on the undulator period, the magnetic field strength, and the electron energy. Thus, a FEL can be tuned over a very wide range by varying the electron kinetic energy and undulator configuration. Free electron lasers are unique in their ability to produce highly coherent radiation at exotic wavelengths that support research in fields as diverse as physics, molecular dynamics, medicine, and materials research.
FEL photoinjectors
The Linac Coherent Light Source (LCLS) at the Stanford Linear Accelerator (SLAC; Menlo Park, CA) is the first FEL to produce hard x-rays with kiloelectron-volt energy. The LCLS uses one third of the 3 km linear accelerator to generate pulses with energy greater than 1 mJ in the range from 500 eV to more than 9 keV with pulse durations of several hundred to less than 4 fs, and repetition rates of 30 to 120 Hz.
Free electron lasers require extremely bright electron beams in order to reach gain saturation in the undulator. A high-brightness electron beam is enabled by using an ultrafast laser amplifier to produce a burst of electrons by illuminating a photocathode in an RF accelerating structure called a photoinjector (see Fig. 1). Control of the ultrafast laser enables precise optimization of the time when the electrons leave the photocathode, tightly synchronizing this pulse to the rest of the electron accelerator. It also allows optimization of the spatial and temporal profile of the electron bunch.
The LCLS uses a photoinjector with a copper photocathode. Copper offers a good compromise between reliability and quantum efficiency when excited with the third harmonic of a Ti:sapphire-based ultrafast amplifier. The amplifier produces multi-millijoule pulses, 1–5 ps in duration at 760 nm. Its output is frequency tripled to 253 nm and relay-imaged several meters to the photoinjector photocathode. The photoinjector laser is operated continuously for weeks at a time and requires exceptionally high stability of the spatial mode, temporal profile, and pulse energy. Part of the infrared (IR) beam is also used as a "laser heater," interacting with the initial electrons to reduce the onset of instabilities in the electron beam.
The laser system is operated in the picosecond regime because the FEL photoinjector runs at an RF frequency of 2856 MHz, corresponding to a cycle time of 350 ps. The laser pulse duration is optimized for minimal energy spread from RF effects and detrimental space-charge interactions between the electrons in the bunch. Specifically, longer pulses (tens of picoseconds) would have higher energy spread due to differences in the RF accelerating gradient during the pulse. Shorter pulses (hundreds of femtoseconds) would produce excessively high electron density and result in a large distribution of transverse and longitudinal momentum.
Seeding a FEL
The new Fermi@Elettra (a free electron laser project at the Elettra Synchrotron in Trieste, Italy; see Fig. 2) also uses a copper photocathode driven by the frequency tripled output of a Ti:sapphire system. This is a custom Coherent amplifier incorporating a regenerative stage followed by two double-pass amplifiers. The system delivers IR pulses with a bandwidth of 10 nm, which are chirped before the harmonic conversion to UV so as to optimize subsequent pulse shaping. The Fermi team has built a temporal shaping setup in the UV based on Fourier-shaping and a piezo-deformable mirror in order to produce a long pulse with an increasing ramp shape. The deformable mirror should allow adaptive optimization of the pulse shape for optimum FEL performance. In the same fashion as LCLS, Fermi also uses part of the IR beam to add incoherent energy spread to the electron beam so as to suppress space-charge instabilities that otherwise can degrade beam quality and limit FEL output (this technique was invented at SLAC).The main goal of seeding is to achieve higher wavelength precision and shot-to-shot reproducibility with respect to the SASE scheme. The first successful seeding tests at Fermi were carried out on Dec 13, 2010, with a goal of a fully functioning user facility by the end of 2011.
To provide FEL tunability the seed laser has to be widely tunable in the UV, so the Fermi team uses the upconverted UV output of an IR optical parametric amplifier that produces 100 MW of peak power in the wavelength range 200–260 nm.
With pulses shorter than 100 fs, the synchronization of the laser and accelerator is critical to achieve efficient seeding. Given the size of the facility and the large distance between the subsystems needing synchronization, conventional RF-based synchronization would not enable the required <50 fs rms timing jitter. Instead, a fiber laser at 157 MHz acts as a master oscillator to provide the overall timing to all key components around the Fermi facility via stabilized fiber links.
External synchronization
The timing of the FEL and ultrafast laser must also be synchronized for the pump-probe experiments. At SLAC, these experiments are carried out in one of six hutches where the FEL beam can interact on the sample with one of several ultrafast amplifiers and tunable optical parametric amplifiers (OPAs). The timing of the lasers is controlled by a timing and synchronization distribution network developed by Lawrence Berkeley National Laboratory (LBNL).
Even if the lasers are locked to the timing system of the facility, there is significant jitter between the RF pulses and the arrival of the final FEL output pulse, so this approach delivers timing stability of only ~1 ps. With FELs and ultrafast amplifiers capable of producing sub-5 fs and sub-35 fs pulses, respectively, this stability compromises the overall temporal resolution. An additional feedback loop therefore measures directly the arrival time of the FEL electron bunches and uses this to correct the raw pump-probe data. The system individually bins each pump-probe data point into its correct time-delay window (see Fig. 4). The end result is an effective jitter of less than 100 fs rms with the prospect of improving to 50 fs.Typical pump-probe experiments
While Fermi is still being brought on-line, SLAC is running two 12-hour shifts, five days per week for user experiments. About 50% of these are pump-probe experiments, which come in two basic varieties: pump with the FEL and probe with an ultrafast laser, or vice versa.The experiments are extraordinarily diverse. The x-ray output of the FEL, for instance, can manipulate or probe inner electrons from atoms and molecules, enabling the ultrafast laser to probe any rearrangement of the residual structure in real time. And in biochemistry, an ultrafast pulse may excite an optical transition while the subsequent x-ray pulse looks for structural changes in x-ray diffraction—a technique intended for exploring the unfolding of proteins. In materials science, a sample is hit with an ultrafast pulse to cause lattice compression, while the x-ray pulse observes the compression and relaxation in real time. In another example an ultrafast laser aligns the dipoles in a stream of molecules (such as nitrogen), which is then dissociated with x-rays. This enables the spatial ejection of the fragment to be studied by a time of flight detector (see Fig. 5).
Alan Fry | Staff Scientist, SLAC National Accelerator Laboratory
Alan Fry is staff scientist and LCLS Laser Science Department deputy at the SLAC National Accelerator Laboratory (Menlo Park, CA).
Miltcho Danailov | Leading Scientist, Laser Laboratory at Sincrotrone-Trieste
Miltcho Danailov is leading scientist, Laser Laboratory at Sincrotrone-Trieste (Trieste, Italy).
Marco Arrigoni
Marco Arrigoni is vice president of marketing at Light Conversion (Vilnius, Lithuania). He previously served as director of marketing at Coherent (Santa Clara, CA) from 2007 through 2023.