Over the past few years, time-resolved (“lifetime”) fluorescence spectroscopy and imaging (see “How they work,” bottom of page) have moved steadily toward fulfilling their promise of clinical benefit.1 Time-resolved fluorescence has recently been studied for characterization of atherosclerotic plaques2 and carotid arteries,3 in vivo detection of radiation-induced necrotic changes to the brain,4 and diagnosis of rheumatoid arthritis5 and oral cancer6—all in real time and without the use of exogenous agents.
The many advantages of lifetime fluorescence for clinical applications are evident in the example of intraoperative image-guided cancer surgery.
Cancer surgery and image guidance
Surgery is the preferred treatment for most cancers, and currently, tumor removal relies on a surgeon’s ability to detect anomalies visually and by palpation—skills that challenge even the most experienced practitioners.
Standard (non-time-resolved) fluorescence-guided surgery (FGS) is an approach in which the patient is injected with a contrast agent that has affinity for diseased cells. The cancerous tissues then glow when illuminated by light of a corresponding wavelength, giving surgeons a better chance of removing all diseased tissue and patients a decreased chance of metastasis.
FGS is a significant advance over the current standard of care. Its progress has been hampered, however, by a dearth of fluorophores approved for use in humans, even though development of fluorescent markers is on the rise—as is demand for image-guided surgical systems.
In 2010, Intuitive Surgical (Sunnyvale, CA) introduced FGS as an add-on for its da Vinci Surgical System via a module called FireFly, which uses FDA-approved indocyanine green (ICG) to provide near-infrared fluorescence (NIRF) imaging of blood flow and tissue perfusion (see Fig. 1). Released in 2000, da Vinci is used for more procedures than any other robotic surgery setup. The module is an important addition because robotic surgery removes the possibility of tactile feedback, leaving visualization as the only means of assessment. But because ICG is not a molecularly targeted probe, it lacks specificity for critical cancer assessment and cannot provide quantitative assessment of tumor margins. And the fact that ICG-based imaging requires that lights in an operating room be turned off means it does not integrate seamlessly into workflow.Searching for a more robust means of image guidance, Intuitive Surgical turned to time-resolved autofluorescence. As a label-free technique, time-resolved fluorescence spectroscopy (TRFS) relies on endogenous fluorophores as opposed to injected biomarkers, and they overcome limitations of steady-state fluorescence techniques studied for diagnosing oral cancer. The time-resolved contrast mechanism enables discrimination among fluorophores that have overlapping spectra, and it provides information about the molecular composition of tissue by revealing changes to the structural and metabolic characteristics resulting from cancer. Specifically, the approach has been demonstrated to distinguish tumors from normal tissues in head and neck cancer.
Working with Laura Marcu, Ph.D., of the University of California Davis, a pioneering researcher in the field of time-resolved fluorescence, Intuitive Surgical recently undertook the first-in-human study involving integration of autofluorescence in robotic surgery. The work studied the use of time-resolved tissue diagnostics for real-time surgical guidance.
Based on previous work by the Marcu lab on scanning multispectral time-resolved fluorescence spectroscopy (ms-TRFS) and in collaboration with Dr. Gregory Farwell of the UC Davis School of Medicine, the group integrated an ms-TRFS subsystem into the da Vinci system using a customized fiber-optic probe. They evaluated the integrated system’s ability to complement visual inspection in vivo, first in swine models and then in human subjects with oral cancer undergoing transoral robotic surgery (TORS).7
Design and integration of ms-TRFS
The work involved development of a customized fiber-optic probe that the team integrated into the working channel of the da Vinci system’s multi-jointed 5FR EndoWrist Introducer, which provides great dexterity for operation in tight surgical spaces. The probe incorporates a single multimode silica fiber with a 400-μm-diameter core to supply fluorescence excitation and collection.
All components involved in performing ms-TRFS measurements are housed in a compact cart designed to integrate well with operating room procedures. A micro Q-switched laser, frequency-tripled to 355 nm with a 2 kHz repetition rate, supplies fluorescence excitation. The optical probe collects the fluorescence signal using a wavelength selection module (WSM) incorporating dichroic and bandpass filters that simultaneously resolve fluorescence emission into four spectral channels: 390/40 nm, 466/40 nm, 542/50 nm, and 629/53 nm. A 445 nm continuous-wave solid-state laser coupled into the 466/40 nm channel injects a 3 mW aiming beam onto the same path as the excitation beam. Optical fibers of differing lengths (to act as delays) couple with the four channels into a single detector (a microchannel plate photomultiplier tube). The PMT detects the fluorescence signals and converts them to electrical signal, which an RF amplifier processes before sending to a high-speed digitizer for sampling at 80 ps time intervals. The system modulates the photodetector’s bias voltage (and gain) in real time to handle signal changes resulting from differing signal-generation abilities of particular tissue types, as well as differences in probe-to-tissue distance. An AC-coupled amplifier filters out autofluorescence induced by the aiming beam.A computer receives freehand scanning data from the optical fiber-probe along with white-light video from a frame grabber that processes the endoscopy input. It correlates the locations of these inputs and sends them to the display with ms-TRFS data—depicting biochemical properties of tissue as fluorescence parameters—overlaid onto the video stream (see image above).
Application for oral cancer surgery
For the TORS application, the team designed the system to collect and analyze ms-TRFS data from the oropharynx in real time both before and after tumor excision.
Two features serve to facilitate data acquisition during surgery. The first is a method that optimizes signal amplitude against the dynamic range of the digitizer. This scheme relies on a closed-loop control algorithm8 (see Fig. 2) to provide feedback to a high-voltage power supply, which then determines photon multiplication gain and dynamically alters signal amplitude. The second is a foot pedal that allows the surgeon to trigger both laser excitation and data acquisition.Control conferred by the foot pedal helps the surgeon prevent photochemical damage to tissue. The system tracks the areas exposed to laser light and computes exposure. The exposed locations are identified in the ms-TRFS augmented video stream, and based on light pulse characteristics, the system determines a maximum permissible exposure (MPE) value. It also tracks a cumulative exposure for each pixel in the field of view, and when the cumulative level reaches 50% of the MPE, corresponding pixels are marked to warn the surgeon to avoid those areas.
In practice, the ms-TRFS approach was able to differentiate among carcinoma in situ (including carcinoma over lymphoid tissue) and normal tissue.
Significance
While earlier fiber-optic-based time-resolved fluorescence instruments demonstrated potential to differentiate between cancerous and normal tissues in the oral cavity, they could not provide either real-time tissue biochemistry feedback or fluorescence parameters on the tissue surface. They were also unable to trace-back evaluated areas—a function important not only for intraoperative guidance, but also for further validation with histopathology analysis. This work demonstrated for the first time continuous real-time visualization of areas interrogated by a fiber-optic probe and streamed to a surgeon, and the ability to generate diagnostic contrast based on autofluorescence properties.
The work shows that integration of label-free ms-TRFS into the da Vinci had no negative effect on either system. It allowed recording of robust data during TORS procedures in human patients, effectively providing contrast in real time and allowing surgeons to dynamically incorporate this information during procedures. The ms-TRFS system’s high dynamic range (enabled by dynamic control of gain applied to the detector) ensures robust data acquisition, regardless of changes in quantum efficiency or light-collection geometry.
The only limitation of note is the potential for tissue movement to degrade acquired data. While this can be effectively controlled in TORS, laparoscopic procedures may provide a greater challenge. However, the team thinks that development of methods to detect motion and/or tissue deformation—and correspondingly alter output—may sufficiently address the problem. Moreover, they point out, surgeons rely on the assessment of relatively small areas, and such limited areas can be imaged without motion blurring.
Future plans include automatic disabling of the excitation source so that regions that have reached the MPE threshold cannot be accidentally overexposed.
The integration of label-free, real-time assessment and visualization of tissue biochemistry into robotic surgery promises to help prevent positive tumor margins (which is recognized as a key quality metric) and to improve intraoperative decision-making—all without demanding modification of current clinical protocols. What’s more, the work points to eventual clinical translation of other point-scanning optical techniques, including spectroscopy techniques such as elastic scattering, diffused reflectance, and Raman.
REFERENCES
1. L. Marcu, BioOptics World, 7, 7, 27–30 (2014).
2. B. Bilen et al., Sci. Rep., 8, 14378 (2018).
3. A. Alfonso-Garcia, A. K. Haudenschild, and L. Marcu, Biomed. Opt. Express, 9, 9, 4064–4076 (2018).
4. B. A. Hartl et al., Biomed. Opt. Express, 9, 8, 3559–3580 (2018).
5. P. Z˙uchowski, M. Waszczak-Jeka, S. Kudlicki, and S. Jeka, Reumatología, 57, 1, 45–49 (2019).
6. D.G. Farwell et al., Arch. Otolaryngol. Head Neck Surg., 136, 2, 126–133 (2010).
7. D. Gorpas et al., Sci. Rep., 9, 1187 (2019).
8. D. Ma, J. Bec, D. Gorpas, D. R. Yankelevich, and L. Marcu, Biomed. Opt. Express, 6, 987–1002 (2015).
How they work
Label-free time-resolved fluorescence techniques are based on the fact that many endogenous biomolecules generate autofluorescence. The span of time between absorption of a photon and subsequent emission, or excited-state “lifetime,” is characteristic for every molecule. These lifetimes serve as contrast agents and are measurable. Time-resolved fluorescence spectroscopy (TRFS) measures the temporal dynamics of autofluorescence at high spectral resolution, while fluorescence lifetime imaging microscopy (FLIM) maps the concentration and distribution of constituent fluorophores to depict the biochemistry of a tissue as a fluorescence emission profile.
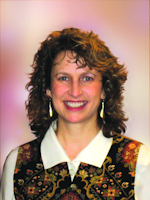
Barbara Gefvert | Editor-in-Chief, BioOptics World (2008-2020)
Barbara G. Gefvert has been a science and technology editor and writer since 1987, and served as editor in chief on multiple publications, including Sensors magazine for nearly a decade.