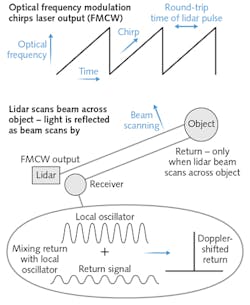
Early on in the development of autonomous cars, design engineers chose pulsed lidar systems emitting at 905 nm because such systems were commercially available to plug into their test vehicles. However, today’s 905 nm pulsed lidar has important limits: the high cost of mechanical scanning, interference from solar glare and other light sources, and eye-safety power restrictions that limit lidar range to 60–100 m. Shifting to the retinal-safe 1550 nm band allows pulse powers high enough to range from 200 to 300 m, and advances in solid-state scanning should reduce costs. Time-of-flight pulsed lasers remain the industry leaders, but they still may not meet the tough requirements to achieve true automotive autonomy.
Now, coherent lidar is entering the race for automotive applications. The leading approach is frequency-modulated continuous-wave (FMCW) lidar, an optical version of the inexpensive FMCW coherent microwave radars now used as safety accessories in some cars. Continuous-wave operation avoids the eye hazards of high peak powers that limit present pulsed lidar ranges. Coherent detection is also much more sensitive than direct detection and offers better performance, including single-pulse velocity measurement and immunity to interference from solar glare and other light sources—including lidars used by other cars. However, FMCW lidar faces serious challenges.
Pulsed and coherent lidars
Pulsed lidars are simple time-of-flight ranging systems. They measure distance by recording the time between emission of a short pulse and reception of reflected light, and in the process measuring the angle of the reflected light to measure object position. However, measuring velocity toward the lidar requires firing multiple pulses and dividing the change in distance between pulses by the interval between pulses. This time adds up in an environment full of moving vehicles.
Coherent lidars locate objects by mixing light reflected back from the object with light from the coherent laser transmitter. Motion of the object toward or away from the lidar Doppler-shifts the return signal, so mixing it with the local oscillator signal directly measures velocity in the line of sight with a single observation. As with pulsed lidar, the angle of the return signal measures the direction of the object from the transmitter. Analyzing the intermediate frequency generated by mixing the lidar return with the output signal measures object distance, and combining that with direction and velocity gives a three-dimensional location as well as velocity. “Coherent detection allows for a lot more signal processing to do more interesting things,” says Stephen Crouch, cofounder and CTO of Blackmore (Bozeman, MT).
FMCW lidar measures distance by repeatedly linearly chirping frequency of the continuous laser beam (see Fig. 1). Typically, the chirp rises for a time longer than the light takes to reach the object, which then reflects the beam as it scans past. When the return is mixed with the local oscillator, a coherent detector mixes the signals and measures the difference frequency, which tells how much the frequency changed while the reflected light made its round trip to the object. Multiplying that interval by the chirp speeds yields the distance. Further processing extracts the Doppler shift to yield the object’s velocity relative to the lidar, which is critical to keep self-driving cars aware of other vehicles moving around them.
A big advantage of coherent detection for signal processing is that it amplifies only light coherent with the local oscillator signal, says Greg Smolka, vice president of business development at Insight LiDAR (Lafayette, CO). “Your detector is looking for an exact match that comes back coherent with the lidar beam,” he notes. The mutually coherent light is what is amplified—light that does not match the local oscillator is not detected, blocking noise from sunlight, artificial lights, or lidars on other cars. In contrast, pulsed lidar cannot screen out the incoherent light, which can interfere with the return.
Existing FMCW lidars are limited to coherence lengths around 100 m, which could restrict their range to about 50 m—a serious limitation. Current pulsed lidars with ranges of 60 to 100 m are adequate for urban robotaxis moving at a leisurely pace. However, cars at highway speeds need ranges of 200 to 300 m to stop in time to avoid collisions.
Efforts are underway to overcome that limit. One option is advanced signal processing, which Taehwan Kim of the University of California at Berkeley (Berkeley, CA) says might extend FMCW lidar range by a factor of 10. Coherent lidar returns produce sharp peaks for objects within the laser’s coherence length, but broaden to a Lorentzian shape beyond it (see Fig. 2). Processing such lidar returns with conventional fast Fourier transforms degrades their precision and sensitivity, but Kim has reported improvements beyond the coherence length by processing them with Lorentzian least-squares fitting. The goal is to stretch FMCW lidar ranges far enough for use at highway speeds.Photonic integration
Another concern about FMCW systems is their greater cost and complexity than time-of-flight pulsed lidar. “In many ways, FMCW lidar is more difficult, with more stringent requirements on the laser source,” says Smolka. Insight LiDAR has been working on photonic integration of FMCW lidars for two and a half years, including swept-source lasers, on-chip amplification, and detectors that allow ranging objects with 10% reflectivity to at least 200 m.
The company is also pushing for the performance that would be needed in a world full of fast-moving robotic vehicles. “It’s not enough to be able to see something at 200 meters,” says Smolka. “You have to put enough pixels on it to identify what it is, and if it’s something I have to worry about.” To do that, they use solid-state scanning on the fast axis and mechanical scanning on the slow axis to allow high-resolution scans of far-field objects of interest such as pedestrians.
Optical phased arrays are another approach to solid-state scanning that can be used in integrated photonics. They phase-modulate laser light as it passes through waveguides to shape and redirect the beam emerging from the output end of the array. The first report of using integrated silicon photonics to make an optical phased array for FMCW lidar came from a team at the Massachusetts Institute of Technology (MIT; Cambridge, MA) led by Christopher Poulton in 2017. They added the optical phased array to an on-chip balanced detector with edge couplers for both transmitting and receiving light to create a lensless chip-scale FMCW lidar. They chose a 1550 nm laser source to transmit through silicon waveguides and chirped its frequency linearly with frequency first rising, then falling. They reported steering light across a field of 46° × 36°, but their range was limited to only 2 m and its resolution was low. Since then, others have reported better integrated lidar results and longer ranges.
Optical phased arrays are attractive because they have virtually no side lobes and can transmit and receive through a single set of optics, says Mehdi Asghari, CEO of SiLC Technologies (Monrovia, CA), a 20-year veteran of silicon photonics. With a narrow-line laser and low-noise receiver, he says a phased array lidar can reach the 200–300 m range needed for self-driving cars. Adding an indium-phosphide gain chip emitting at 1550 nm and germanium devices to a silicon chip containing the external cavity “beats most expensive complicated lasers,” he adds.
He says the SiLC design can eventually provide eye-safe lidars with ranges to 500 m. They have reached a 100 m range with a 4 mW laser, and think they can reach 200 m with 50 mW. A single laser core in the lidar should suffice for most short-range vehicular applications, and adding more cores could reach further. Multicore lidars could use lasers with different wavelengths to identify what they are measuring. The company plans to sell sensors and processors to lidar makers rather than make their own lidars.
Blackmore has teamed with Sandia National Laboratories (Albuquerque, NM) on a planar phased-array chip that splits light from a 1550 nm laser among waveguides in the plane of a wafer, then directs the light upward to create a steerable optical beam (see Fig. 3). Crouch calls it “a nice collimated optical beam coming up off a credit card.” He says the big advantage of operating at 1550 nm is the availability of sophisticated, high-efficiency components developed for fiber-optic components.Looking forward
FMCW lidar technology is advancing, but it remains challenging. “We’re not going to tell you that we are looking out to 200 meters today with optical phased arrays,” says Crouch. He says phased arrays are a few years out, but is optimistic about the future of automotive applications, adding that “The long game is to get the cost of lidars low.”
Yet others worry that FMCW lidar may be too complex and costly for autonomous vehicles. There are a lot of tradeoffs between time-of-flight and coherent lidar, says Umar Piracha of Imec, an international R&D and innovation hub headquartered in Belgium, which has been working on both types. “FMCW gives you extra gain by mixing the faint return signal with the reference oscillator, making the receiver more sensitive. If you get a tiny amount of power from reflection off a black car far away, coherent detection can amplify the signal to spot it,” he says. That saves money by allowing receivers to use $1 p-i-n silicon detectors instead of costly avalanche photodiodes or silicon photomultipliers needed in pulsed lidars.
Yet Piracha adds that a FMCW lidar “requires a tunable laser with good polarization control and very long coherence length.” He warns of reduced sensitivity from speckle noise and high costs from overall system complexity. “The real question is how applicable and easy it is for use in self-driving cars,” he says. “While time-of-flight systems have seen greater overall interest due to their simplicity, the answer is not really clear.”
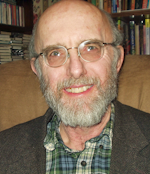
Jeff Hecht | Contributing Editor
Jeff Hecht is a regular contributing editor to Laser Focus World and has been covering the laser industry for 35 years. A prolific book author, Jeff's published works include “Understanding Fiber Optics,” “Understanding Lasers,” “The Laser Guidebook,” and “Beam Weapons: The Next Arms Race.” He also has written books on the histories of lasers and fiber optics, including “City of Light: The Story of Fiber Optics,” and “Beam: The Race to Make the Laser.” Find out more at jeffhecht.com.