Looking to the future of quantum cascade lasers
The first experimental demonstration of the quantum cascade laser (QCL) was conducted in 1994 by a team at Bell Labs, led by Federico Capasso, the Robert Wallace Professor of Applied Physics at Harvard University. He noted that after almost 30 years from the invention of QCLs, they “are the only widely wavelength-tailorable and tunable light sources in the mid-infrared spectrum, and combined with compactness and room-temperature operation, have opened up a broad range of applications in science and technology, impacting spectroscopy, atmospheric chemistry for climate change studies, trace gas analysis, pollution monitoring, chemical sensing, diagnostics in sectors such as medicine, materials, and combustion, and military applications such as infrared countermeasures.” To date, lasing wavelengths have been demonstrated from as short as 2.63 μm to as long as 250 μm under standard operating conditions, although most commercially available QCLs tend to be in the 4–12 μm range.
The significance of QCLs is that they can unlock a complete frequency range in the mid-infrared (mid-IR).
Quantum cascade lasers
Traditional semiconductor laser diodes use transitions in the p-n junctions of the semiconductor material to produce light emission. In contrast, in a QCL, the lasing transition occurs between states within a given quantum well. The advantage of this construction is that the electron responsible for the emission of the photon tunnels into the next quantum well and, as a result, multiple photons can be generated by a single electron, thereby making them extremely efficient.
The tunneling from one well to the next gives rise to the concept of “quantum cascade”; the well depths can be engineered by controlling layer depths during the fabrication process to produce the desired wavelength of the lasing transition. The main advantage of this approach is that because the emitted wavelength only depends on the layer thicknesses and not on the constituent materials as with traditional semiconductor lasers, this technology can produce lasers in a very broad spectral range (λ from 3 to 160 µm).
Compared with traditional Fourier-transform infrared (FTIR), mass spectroscopy, and photothermal micro-spectroscopy systems, QCLs have a shorter response time and a much wider tuning range covering both mid- and longwave IR bands. These features allow for faster and more precise compact trace element detectors and gas analyzers, making QCLs ideal for a variety of sensing and spectroscopic applications.
Spectroscopy and the IR fingerprint region
One of the technologies benefiting from the use of QCLs is IR spectroscopy, which for more than a century has been used to chemically characterize substances by absorption or transmission. All covalently bound materials have a unique absorption spectrum in the IR spectrum due to the allowed vibration and rotation modes arising from the molecular structure. The various absorption bands associated with each molecular bond form a unique spectrum—known as a molecular fingerprint (the spectrum in the 5–14 μm range)—in which the vibrational resonances of most liquids, gases, plastics, glasses, and biological tissues, as well as radiation from celestial objects, can be found (see Fig. 1).
Traditionally, IR absorption has been measured using a FTIR spectrometer consisting of a broadband IR light source and a scanning interferometer. QCLs substantially simplify the system by completely removing the interferometer from the setup and replacing the IR light source with a tunable QCL. As a result, QCLs are now widely utilized in chemical analysis and chemical imaging, as they can significantly reduce the cost and complexity of the system.
Furthermore, QCLs are considerably brighter than other coherent sources in the fingerprint region, providing enough brightness for standoff, noncontact sensing of substances at long distances (up to hundreds of meters) with very small optical coupling loss. Additionally, the high brightness of QCL sources results in sufficiently high signal-to-noise ratio (SNR) to eliminate the requirement for high-sensitivity cryogenic detectors and thus the nuisance of a liquid nitrogen supply.
Applications
As a consequence of their small size, low power requirement, and enhanced functionality compared to traditional semiconductor lasers, QCLs are being increasingly used in several application fields (see Fig. 2), including:
Industrial controls (online gas sensing). The high beam quality of QCLs enables long path lengths that are useful for the monitoring of large indoor and outdoor facilities such as transportation terminals, chemical plants, refineries, and military bases. For example, a QCL-based system can scan the air inside of an airport terminal and detect the release of airborne chemical threats. A similar system is used for online monitoring of gases in pipes for process control and the remote, real-time sensing of industrial exhaust stacks for environmental regulation without the need for sampling the gas. For more complex gas molecules that absorb at longer wavelengths, terahertz QCLs are now being commercialized—some emitting in the range of 100 to 150 µm, which provide greater measurement accuracy than with current technologies.
Defense and security. A major defense application of QCLs is missile protection of helicopters or airplanes, commonly known as direct infrared countermeasure (DIRCM) where high-power 4 µm QCLs are used for heat-seeking missile countermeasures. Also, improvised explosive devices (IEDs) are often made of compounds that absorb in the terahertz range, and ruggedized, portable terahertz QCL-based detectors can be continuously scanned ahead of a moving convoy or used to search a public gathering space for threats.
Environmental monitoring. Excessive quantities of pollutants (NOx, CO, NH3, VOC, etc.) emitted into the Earth’s atmosphere by transportation, industry, and agriculture livestock may cause diseases, allergies, and sometimes death of humans; it may also cause harm to other living organisms such as animals and food crops and may damage the natural or built environment. Monitoring and diagnosis of air quality are essential for providing clean and safe air for breathing. In this context, compact (portable) and accurate instruments based on laser absorption spectroscopy are available for real-time detection of several gases (SO2, CO, CO2, NH3, VOC, NOx, CH4, etc.) and are capable of gathering reliable data about global warming.
Healthcare. QCL-based systems are also finding applications in the growing field of medical diagnostics because in the 3–12 μm region, sharp and strong absorption peaks appear, thanks to the fundamental vibrations of various molecules that make up biological tissue. Accordingly, biological tissue can be analyzed by investigating the absorption spectrum (the so-called “fingerprint spectrum”) formed by these molecules, making it possible to identify the constituent molecules and analyze their components. In principle, by using mid-IR absorption spectroscopy, components such as proteins, sugars, and lipids contained in blood or interstitial fluid can be analyzed noninvasively. Therefore, the development of healthcare systems performing health check-ups on-site and in real time without blood sampling is anticipated. For example, trace gases present on a patient’s breath can indicate diabetes, asthma, and other respiratory issues, kidney and liver dysfunction, and other indicators are being discovered regularly. QCL spectroscopy allows chemical analysis of surface layers, such as the characterization of special osteophilic coatings that are applied on the surface of orthopedic implants to improve acceptance of the implants by human tissue.
Automotive. Measurement of nitrous oxide levels in vehicle emissions is becoming increasingly important because of their contribution to global warming. The advantage of using a QCL is the extremely narrow bandwidth of the emitted laser light, where a very high selectivity to the desired measurement component can be achieved. QCL-based gas analyzers are currently available for the direct, simultaneous, real-time measurement of the four relevant nitrogen-containing exhaust gas components (NO, NO2, N2O, and NH3) with high selectivity and sensitivity and no crossover.QCL manufacturing scenario
Nowadays, QCLs are definitely the most versatile mid-IR lasers and also capable of emitting in the terahertz radiation region. Furthermore, QCLs are far more compact and rugged than other mid-IR sources such as OPOs/OPAs. As a result, a QCL is the light source for many applications operating in the mid-IR. The flexibility of QCLs leads to a widely diversified range of products offered by companies as original solutions to achieve the common goal of a small and rugged package. Additionally, each company is characterized by its own business model. For example, Dr. Mathieu Carras, CEO and founder of the French company mirSense, notes that “in order to break the vicious circle of the high price of QCLs and limited market size, we, at mirSense, are investing into full vertical integration from low-cost laser design to miniaturized photoacoustic sensors (typically cm3 scale) directly addressing larger markets.” mirSense manufactures compact, lightweight, and robust solid-state mid-IR QCLs whose structures (active region, waveguide, and distributed feedback) are designed with proprietary modelling tools to ensure high agility in responding to customer needs. They are the only QCL manufacturer to exploit two different semiconductor technologies—the standard indium phosphide (InP)-based technology and the alternative indium arsenide (InAs)-based technology. This allows them to extend the accessible wavelength coverage of QCLs down to 3 µm on one side and up to 20 µm on the other side, which makes them suitable for applications such as defense and gas sensing through molecular spectroscopy (see Fig. 3). The lasers work at room temperature, can be operated in pulsed or continuous-wave (CW) mode, and are tuned by changing the internal QCL temperature or driving current.Lytid, also based in France, has pushed quantum well technologies for sensing to their limit with the state-of-the-art 5 μm mid-IR detector, MIRQWIP, which they claim is the fastest mid-IR photodetector on the market. Applications include high-speed mid-IR optical links, heterodyne instrumentation, and characterization of QCL combs. Lytid also provides a terahertz QCL that exploits inter-sub-band transitions in cascaded quantum well structures to generate terahertz radiation between 60 and 150 μm for imaging, gas spectroscopy, and space applications. The key advantages are direct generation of terahertz photons and high output power. The device also incorporates proprietary compact laser drivers designed for the specific needs of terahertz QCLs as well as a high-performance, highly reliable cryogen-free cooling system.
According to Dr. Antoine Müller, CEO of Alpes Lasers (a Swiss engineering company pioneering advanced light sources), their newly developed frequency comb QCLs are manufactured with specifically engineered spectral dispersion to produce multimode optical outputs comprising broad and powerful frequency combs. These QCL frequency combs (QCL-FC), first demonstrated in 2012 by a team at ETH Zurich in collaboration with Alpes Lasers, are currently the most promising frequency comb devices available in the mid-IR. The mode spacing of both QCL-FC and ultrashort-pulse lasers is given by the cavity length; however, in the case of QCLs, the periodic modulation in the time-domain is of the frequency modulated (FM) rather than amplitude modulated (AM) type and the output power is constant. A QCL-FC is a standalone device that integrates a pump laser and a micro-resonator in its waveguide. This allows QCL-FCs to be significantly more compact compared with previous technologies. All of these attributes provide QCL-FCs with unique characteristics, making them ideal for a wide range of novel applications, including the amine band (centered around 6.02 μm), which is of interest in the pharmaceutical industry (see Fig. 4).In Japan, the multinational Hamamatsu Photonics produces three types of QCLs in the mid-IR range (4–10 μm) for molecular gas analysis and absorption spectroscopy applications. The first is a DFB-pulsed QCL, the technology for which has been developed further and then incorporated. The first is in the wavelength-swept, pulsed QCL, which realizes broad wavelength sweeping by external cavity configuration (see Fig. 5); the second is the DFB-CW QCLs with a built-in lens that provides a collimated beam in a sealed housing butterfly package; and the third is the DFB-CW QCL, which incorporates single-photon resonance-continuum depopulation (SPC) and distributed feedback structures to provide a stable single-mode emission under room temperature.
Thorlabs offers a wide range of quantum (and interband) cascade lasers to access the mid-IR spectral region: both Fabry-Perot QCLs, well suited for medical imaging, illumination, and microscopy applications, and distributed feedback QCLs emitting at a well-defined center wavelength and providing single spatial-mode operation ideal for chemical sensing, optical communications, and other applications.
Challenges
At present, QCLs are still somewhat specialized devices and face two major challenges. Firstly, manufacturing is difficult to optimize as the devices require system designers to pay particular attention to the driver and temperature-control electronics. Current noise from the QCL driver broadens the laser linewidth, which reduces the overall system sensitivity and precision, and temperature change causes the wavelength to drift. To fully realize the precision made possible by QCLs, it is important that they are powered by ultralow-noise drivers and highly stable temperature controllers. Secondly, small batch sizes result in high unit cost.
The enhanced functionality and spectral characteristics associated with QCLs make them an invaluable tool for many applications. The number of markets for QCLs is growing at an ever-increasing rate, as not only scientists and manufacturers, but also end-users, gain more experience with them. Over the next five years, it is reasonable to predict that as awareness of the value of these devices increases, more applications will be created and QCLs will become more readily available and affordable. To this end, and to fully exploit the current momentum, the Mid-IR Alliance has been established by MIRPHAB, a pilot line for prototyping and production of mid-IR chemical sensing devices able to operate in both gas and liquid mediums; it is an EC-funded initiative in a public-private partnership with Photonics21. The Mid-IR Alliance is an industrial group of companies active in mid-IR technologies at all levels of the supply chain, dedicated to the continued success and advancement of mid-IR technologies. The group includes laser and detector manufacturers, developers of mid-IR cameras and sensors, companies manufacturing photonic integrated circuits, software for modeling, and packaging services. The aim is to become the voice of mid-IR technologies and a bridge between end-users and the companies of this high-tech community.
ACKNOWLEDGMENT
MIRPHAB has received funding from the European Union’s Horizon 2020 research and innovation program under the Grant Agreements No 688265; it is an initiative of the Photonics Public Private Partnership.
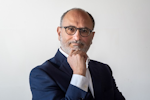
Antonio Raspa | Ultrafast Solid-State Laser Product Manager, Luxinar
Antonio Raspa is ultrafast solid-state laser product manager at Luxinar (Kingston upon Hull, U.K.), and an editorial advisory board member at Laser Focus World. He has more than 35 years of experience with solid-state laser design, photonics components, and fiber optics.
He holds a MSc degree in Electrical Engineering from Politecnico di Milano with a specialization in Quantum Electronics. Before joining Luxinar, he served as senior photonics program manager at the European Photonics Industry Consortium (EPIC; May 2020 – September 2022), preceded by Quanta System (1988-2000) as R&D manager for the development of solid-state laser sources and custom photonics systems for industrial and scientific applications. During this period, he participated, as Ozone LiDAR specialist, to the Italian research program in Antarctica. He then worked at Trumpf (2000-2008) and Rofin-Sinar (2008-2009) as a Product Manager for industrial laser products and processes. In 2009 he returned to Quanta System, organizing and managing a new plant for the production of sterile optical fibers for surgery.
Francesca Moglia | Project Leader, EPIC
Francesca Moglia is a Project Leader at the European Photonics Industry Consortium (EPIC; Brussels, Belgium).