PHOTONIC FRONTIERS: FREQUENCY COMBS: Frequency combs make their way to the masses
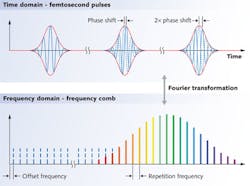
The first optical frequency combs were produced from trains of ultrashort pulses in the late 1970s, but they attracted little attention because their bandwidths were limited and their absolute frequencies could not be measured. Two decades later, a series of advances extended frequency combs to an octave and measured their frequencies precisely. That huge advance in metrology earned John Hall and Theodor Hänsch the 2005 Nobel Prize in physics.
Now frequency combs are being developed for new frontiers in metrology, from calibrating tunable lasers and improving the precision of coherent laser radars to searching for Earth-sized planets around other stars. Meanwhile, development of more compact and robust frequency combs could lead to a wider range of applications, including high-speed telecommunications and space-based instruments.
Types of frequency combs
A frequency comb is a series of evenly spaced optical frequencies, the Fourier transform of a train of short optical pulses. The shorter the pulses, the wider the range of frequencies they contain, and thus the broader the frequency comb’s bandwidth. Femtosecond frequency combs can span an octave. The frequency spacing in the comb equals the pulse repetition rate. Each tooth in the comb is a continuous beam, with power that varies across the spectrum, as shown in Fig. 1.
The first octave-spanning frequency combs were based on Ti:sapphire lasers, which are still widely used. Ti:sapphire lasers directly emit ultrashort pulses at high repetition rates and high peak and average powers. They also have the lowest noise of any frequency comb. But they are bulky, sensitive, and require expensive green pump lasers.
Fiber lasers have lower inherent spectral bandwidth, so they can’t generate pulses as short as Ti:sapphire, but passing their output through nonlinear fibers can stretch their bandwidth to an octave or more. Both erbium- and ytterbium-fiber lasers can produce frequency combs, and both also can be frequency-doubled to shorter wavelengths. Although they can’t match the peak power or repetition rate of Ti:sapphire, fiber lasers are easier to use, less expensive, and more robust. That combination makes fiber lasers more attractive for applications outside the laboratory. And they are being developed for zero-gravity experiments in space.
Microresonator frequency combs
Frequency combs also can be generated by four-wave mixing in a high-Q-factor microresonator. As in an optical parametric oscillator (OPO), pairs of photons from a pump laser combine in a nonlinear material to produce two photons with the same total energy, like the pump and idler in an OPO. However, the comb-generation process uses a tunable continuous beam to pump a microring resonator that oscillates on multiple whispering-gallery modes.
Typically the pump beam is amplified before being coupled into the microresonator, where it generates intensities of gigawatts per square centimeter. That triggers degenerate four-wave mixing, which produces a pair of frequencies, one above the pump line and one below, as shown in Fig. 2. In principle, that shift can be by any amount, but in the microresonator only frequencies matching one of the whispering-gallery modes are amplified, producing lines in a frequency comb, which may be offset by one, two, or more steps from the pump. The additional comb lines then interact with each other by nondegenerate four-wave mixing to produce other comb lines, as shown in Fig. 2, producing a broader frequency comb.The maximum width of a microresonator comb is limited by chromatic dispersion in the resonator material. The four-wave mixing process produces lines that are sidebands, equally offset in frequency. However, any chromatic dispersion present in the nonlinear material causes the whispering-gallery modes to shift with wavelength, so they drift away from the sideband lines as the wavelength changes, reducing the line strength.1
Tobias Kippenberg, now at the Swiss Federal Institute of Technology Lausanne (Lausanne, Switzerland), and colleagues produced the first broadband microresonator frequency combs in 2007.2 The resonator modes in that experiment were 850 GHz apart, a frequency too high for processing by the microwave electronics needed to measure optical frequency. Developers now are pushing to reduce microresonator combs with line spacing of tens of gigahertz, compatible with microwave electronics, says Scott Diddams of the National Institute of Standards and Technology (Boulder, CO).
Developers dream of putting a microresonator comb generator onto a chip, says Diddams, and are exploring many possible designs. Silicon nitride is attractive because it can be fabricated photolithographically on silicon and requires little post-processing. Figure 3 shows one possible SiN structure, along with three other possibilities: silica waveguides on a glass chip, an ultrahigh-Q toroidal silica resonator on silicon, and an ultrahigh-Q millimeter-sized crystal resonator.Yet microresonator frequency combs may not be able to match the low noise of laser combs. Atomic motion at room temperature can make microresonators vibrate, inducing thermal noise into their output. Researchers now are trying to understand the noise limitations of microresonator combs and to develop ways to control them.
Growing diversity of applications
The first frequency combs made a huge change in the exacting process of connecting microwave atomic clocks to optical frequencies. Before combs, NIST needed a large laboratory full of equipment for the task, Diddams recalls. Ti:sapphire frequency combs shrink the setup to a square meter, and erbium-fiber combs reduced size somewhat further and greatly improved the robustness of experiments.
Now the applications are multiplying, as Nathan Newbury of NIST (Boulder, CO) described in the April 2011 Nature Photonics.3 At the top of his list is frequency measurement of optical clocks, with accuracy of one part in 1017 possible by locking a laser comb to the transition being measured. Combs also can calibrate tunable lasers a thousand times more precisely than conventional etalons or gas cells. That precision comes at a cost in complexity, but developers are working on smaller and simpler comb sources.
The calibration application most likely to make future headlines is in astronomy. The search for planets outside the solar system requires detecting tiny Doppler shifts caused by an Earth-sized planet orbiting a star. Frequency combs could calibrate stellar spectra three to four orders of magnitude better than conventional techniques, Diddams says, without needing the extreme precision for atomic clocks. Reducing comb cost and complexity could pay huge dividends for astronomy.
Combs also could provide a new class of spectroscopic sources. Benefits would include “a spectral coverage potentially larger than available using tunable lasers, a collimated single-mode beam, and teeth that can be coupled to a matched cavity for long effective path lengths,” Newbury writes. Taking full advantage of frequency combs requires resolving individual teeth after the light interacts with the sample, but that has already been demonstrated.
Phase coherence and broad bandwidth make combs attractive for transferring frequency and time signals precisely to distant sites. Frequency signals can be sent through hundreds of kilometers of Doppler-compensated fiber with uncertainty of one part in 1019, but time signals from optical clocks are harder to transfer and the feat has yet to be demonstrated. Optical combs could improve precision of laser ranging, either by using the comb as the light source or by using it to measure the spectrum of a conventional coherent lidar. Combs also can generate microwaves with phase noise much lower than from conventional microwave oscillators, promising better radar and interferometric measurements. And combs could even synthesize arbitrary optical waveforms for research by modulating the phase amplitude of individual teeth.
In recent months, two groups have demonstrated that relatively narrowband frequency combs can transmit high-speed optical data for communications. At CLEO in May 2011, Jacob Levy of Cornell University (Ithaca, NY) and colleagues reported transmitting 10 Gbit/s on each of six lines from a microresonator frequency comb. They said that the technique could be extended to an integrated system generating data rates above 1 Tbit/s.4 But they were soon outdone by David Hillerkuss and colleagues at the Karlsruhe Institute of Technology (Karlsruhe, Germany), who built up a single super-carrier by combining 325 teeth of an erbium-fiber comb spanning 1533 to 1565 nm. That allowed them to transmit 26 Tbit/s through 50 km of fiber.5
Matching technology to applications
Formidable challenges remain. The technology is young. Both frequency combs and their applications are largely in the research stage, and developers are working to match their capabilities with application requirements. “Each application has its own requirements,” says Newbury. Some applications are extremely demanding, but he says others “probably don’t need a comb stabilized to the best optical clock in the world.” Astronomy combs are a good example. They don’t need a 17-digit accuracy to spot an Earth-like planet orbiting another star, but that feat would make headlines. The challenge is to develop combs rugged enough to operate in space.And that challenge is being met. Menlo Systems (Garching, Germany), the only company that produces a complete frequency-comb system commercially, has delivered a fully phaselocked frequency comb system to the Center of Applied Space Technology and Microgravity at the University of Bremen (Bremen, Germany).6 That system is playing an important role in experiments studying atom interferometry, in which the instrument drops 120 m in a drop tower at Bremen, shown in Fig. 4. The fiber laser not only survives microgravity, it withstands deceleration by a mound of styrofoam beads at the base of the tower. Menlo Systems has adopted features from the drop-tower system in its standard frequency comb systems, and is developing a special version to meet the more stringent requirements for operation in space.
REFERENCES
1. T.J. Kippenberg, R. Holzwarth, and S. Diddam, “Microresonator-based optical frequency combs,” Science, 332, 555 (Apr. 29, 2011).
2. P. Del’Haye et al., “Optical frequency comb generation from a monolithic microresonator,” Nature, 450, 1214–1217 (Dec. 20, 2007); doi:10.1038/nature06401.
3. N. Newbury, “Searching for applications with a fine-tooth comb,” Nature Photon., 5, 186–188 (April 2011).
4. J.S. Levy et al., “High-Performance Silicon-Based Multiple Wavelength Source”; http://arxiv.org/pdf/1106.2834.
5. D. Hillerkuss et al., “26 Tbit s21 line-rate super-channel transmission utilizing all-optical fast Fourier transform processing,” Nature Photon., 5, 64 (June 2011); doi:10.1038/ NPHOTON.2011.74.
6. http://www.zarm.uni-bremen.de/space-science/fundamental-physics/projects/primus.html
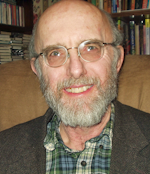
Jeff Hecht | Contributing Editor
Jeff Hecht is a regular contributing editor to Laser Focus World and has been covering the laser industry for 35 years. A prolific book author, Jeff's published works include “Understanding Fiber Optics,” “Understanding Lasers,” “The Laser Guidebook,” and “Beam Weapons: The Next Arms Race.” He also has written books on the histories of lasers and fiber optics, including “City of Light: The Story of Fiber Optics,” and “Beam: The Race to Make the Laser.” Find out more at jeffhecht.com.