FIBER LASERS: Two-micron thulium-doped fiber lasers achieve 10 kW peak power
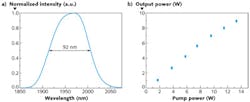
SHIBIN JIANG
The slope efficiency of a thulium (Tm) doped fiber laser can exceed the Stokes limit, enabling a quantum efficiency near 200% for the 3F4→3H6 transition because of the so-called cross-relaxation energy transfer between Tm ions. Using our highly Tm3+-doped (3–7 wt%) silicate glass fibers fabricated by the rod-in-tube technique, laser gain fibers with high slope efficiency of 68.2% and a gain per unit length of >2 dB/cm have allowed us to demonstrate Q-switched fiber lasers and modelocked fiber lasers operating from 1.9–2.1 μm wavelengths based on Tm-doped silicate fibers with peak power levels up to 10 kW.
One popular application of a 2 μm fiber laser with high peak power and high beam quality (from a singlemode fiber) is as a pump source for mid-infrared (mid-IR) frequency generation via nonlinear optical conversion. Another emerging application is for mid-IR supercontinuum generation.
Heavily Tm3+-doped silicate fibers
Compared to their 1 μm and 1.55 μm counterparts, 2 μm laser sources are more favorable pump sources for mid-IR generation for several reasons. First, quantum defects are lower at 2 μm, yielding higher quantum efficiency for generating mid-IR wavelengths. Second, many nonlinear crystals used for mid-IR generation are not transparent or have a much higher absorption at pump wavelengths shorter than 2 μm. Third, dispersion is too high at shorter wavelengths in some nonlinear crystals to achieve phase-matching for nonlinear parametric processes. And finally, 2 μm fibers can have larger core sizes and higher nonlinear thresholds, enabling higher-power 2 μm lasers and consequently, higher-power mid-IR output.
To date, many different glass host materials have been used to fabricate Tm3+-doped optical fibers for 2 μm laser operation, including silica and nonsilica glass fibers such as germanate- and tellurite-based types.1-3 It is well known that the doping concentration of rare-earth ions in silica fiber is limited due to the intrinsic glass network structure. Various approaches have been developed to increase the doping concentration including co-doping with such oxides as Al2O3, B2O3, and P2O5 and by using nanoparticle technology. The highest doping level in silica glass is limited to approximately 2 wt%. Due to the limited Tm3+-doping concentration, quantum efficiency of Tm3+-doped silica fiber lasers is also limited.
Fortunately, the less defined glass network of multicomponent nonsilica glasses permit a higher doping concentration of Tm3+ ions, which in turn permits high pump absorption over a relatively short length of active fiber and allows taking advantage of a 2-for-1 cross-relaxation process in the heavily doped Tm3+ system. AdValue Photonics has developed a proprietary technology to fabricate heavily Tm3+-doped (approximately 3-7 wt%) multicomponent silicate glass and fiber. Because the main glass network is silicon dioxide (SiO2)—the same material as standard silica glass fiber—the doped fiber has improved mechanical strength and better compatibility with silica fiber than other multicomponent glass fibers for more robust fusion splicing between the active fibers and standard passive silica fibers.
Both singlemode and large-mode-area (LMA) double-cladding silicate glass fibers are fabricated using rod-in-tube fiber drawing techniques. The inner cladding of these active fibers is surrounded by a layer of outer cladding silicate glass with numerical aperture (NA) as large as 0.6 to confine the multimode pump laser beam in the inner cladding region. The outer cladding allows for higher-power-handling capability than standard polymer-based double-cladding silica fibers.
A low-index silicate rod is usually inserted into the inner cladding for the enhancement of cladding pump absorption. In a similar way, we also fabricate polarization-maintaining heavily Tm3+-doped silicate glass fibers for cladding-pumped laser operation with slope efficiency values greater than 68% (see Fig. 1).
Q-switched single-frequency thulium fiber lasers
In 2011, AdValue Photonics demonstrated the first all-fiber Q-switched single-frequency laser at a wavelength near 2 μm, which was based on polarization modulation of a short-cavity fiber laser using stress-induced birefringence.4-6 Single-frequency laser operation in the Q-switched mode was confirmed using an in-house fiber-based scanning Fabry-Perot interferometer and the spectral linewidth was measured to be Fourier-transform limited.
Today, we are generating kilowatt-level single-frequency laser pulses from an all-fiber Tm3+-doped source using a singlemode Tm3+-doped fiber amplifier (7.8 μm fiber core, NA = 0.153) to boost the output power of those Q-switched pulses.7 Because of its high pump absorption and high laser gain, tens-of-centimeters-long active fiber is sufficient to build the 2 μm fiber amplifier. For a 20-cm-long segment of active fiber, overall slope efficiency of the amplified laser pulses as a function of total pump power is around 64% at 50 kHz and 40% at a 10 kHz repetition rate.
Multi-kilowatt peak power is possible at low repetition rates (10 kHz and below) with no reduction in pulsewidth evident for the amplifier. The short length of the active fiber suppresses many nonlinear effects; in addition, the short duration of the single-frequency pulses prevents the onset of stimulated Brillouin scattering not only in the fiber amplifier, but also in the longer section of passive fiber after the amplifier.
Q-switched thulium fiber amplifiers
The Q-switched seed laser used in our experiments is an all-fiber actively Q-switched Tm-doped fiber laser. The seed operates at 1950 nm and the repetition rate is tunable from 1 to 100 kHz. At a 10 kHz repetition rate, average power is 30 mW and pulsewidth is approximately 30 ns. In order to achieve higher pulse energy, a preamplifier is applied before the power amplifier.
When the output power from the preamplifier is 200 mW at 10 kHz, the pulsewidth is 26.4 ns. The spectrum of the amplified pulse at 50 kHz has the same width as the seed laser at 10 kHz, but as the repetition rate decreases (the pump power for the preamplifier remains unchanged), the spectrum width becomes broader due to the higher peak power for the lower repetition rate.
The power amplifier uses a 55-cm-long Tm3+-doped (5 wt%) polarization-maintaining fiber with core and inner cladding diameters of 21 μm and 127 μm, respectively, and a core NA of 0.08 that was designed to match the (2+1)×1 pump combiner of the output fiber (20 μm core diameter and 0.08 NA). With an input of 20 μJ pulses at 10 kHz, the slope efficiency of the power amplifier output is about 40% and the pulsewidth is 21.4 ns at the maximum output (see Fig. 2).The laser spectrum after the power amplifier with 16 W absorbed pump power as verified by a spectrum analyzer at different repetition rates showed very good amplified stimulated emission (ASE) suppression for pulses at 40 kHz. The ASE starts accumulating at 20 kHz and at 10 kHz becomes much stronger but is still 40 dB below the pulses. The great ASE suppression is attributed to the short large-core highly Tm-doped silicate fiber. Based on the spectrum we measured at 10 kHz, the calculated ASE power accounts for less than 5% of the total power; therefore, the maximum pulse energy we extract from the amplifier is approximately 600 μJ with a peak power up to 28 kW.
Modelocked fiber lasers
The key element used to start and maintain modelocking operation of a modelocked Tm3+-doped silicate glass fiber laser was a resonant antimonide (Sb)-based semiconductor saturable absorber mirror or SESAM (see Fig. 3).8 The laser has a linear cavity formed by the SESAM, a pump combiner, a 30-cm-long double-clad Tm3+-doped silicate fiber with 10 μm core diameter and a fiber loop mirror. A multimode pump combiner delivers pump light to the Tm3+-doped fiber from a 798 nm laser diode with a 0.22 NA, 105 μm/125 μm fiber pigtail. The fiber loop mirror has estimated reflectivity of approximately 90% at 2 μm.Due to the difference in refractive index between passive fibers (commercial singlemode silica fibers) and the Tm3+-doped silicate fiber, we angle-cleave and fusion-splice the two different kinds of fibers to prevent any spurious reflection that could be detrimental to modelocking operation. The fiber output end is also angle-cleaved to eliminate backreflection. Low-index recoating is performed at the splice between the combiner output fiber and the Tm3+-doped silicate fiber to efficiently couple the pump light and the input end of the pump combiner signal fiber is directly butt-coupled to the SESAM (see Fig. 3).
As pump power increases, the fiber laser operates at several stages. First, continuous-wave (CW) operation is obtained after laser threshold is reached. Then Q-switching or Q-switched modelocking is observed. Further increasing the pump power achieves CW modelocking. At even higher pump power, the laser can experience a transition from single-pulse to a multipulse regime due to the upper limit for pulse energy of the soliton fiber laser that is determined by the laser cavity parameters such as saturation energy of the saturable absorber, modulation depth, cavity dispersion, and self-phase modulation.
Beyond this pulse energy limit, multiple pulses with reduced energy and longer duration are favored over a single short pulse in terms of modelocking stability. For maximum average output power of 10 mW in the single-pulse regime, the pulse energy is around 0.76 nJ. Pulsewidth as characterized with a commercial autocorrelator showed a full-width half-maximum (FWHM) of about 340 fs.We have developed several highly Tm-doped silicate fibers for modelocked pulse amplification. For one of those fiber types, we amplified the modelocked seed pulses with a 45 cm length of Tm-doped silicate fiber with 22 μm core and 0.06 NA (see Fig. 4). Without pulse distortion, the maximum pulse energy is 46 nJ and the corresponding pulsewidth is 1.8 ps for a peak power that exceeds 25 kW. While this was a hero experiment, our current high-power modelocked fiber laser products using this configuration routinely feature greater than 10 kW peak power.
REFERENCES
1. S.D. Jackson and T.A. King, Opt. Lett., 23, 18, 1462–1464 (1998).
2. J. Wu et al., IEEE Photon. Technol. Lett., 18, 334–336 (2006).
3. J. Wu et al., Appl. Phys. Lett., 87, 211118–211120 (2005).
4. J. Geng et al., Opt. Lett., 34, 23, 3713–3715 (2009).
5. Y. Kaneda et al., Proc. Advanced Solid-State Photon., Vol. 94 of 2004 OSA Trends in Optics and Photonics Series, post-deadline paper PD5, Santa Fe, NM (2004).
6. M. Leigh et al., Opt. Lett., 32, 8, 897–899 (2007).
7. J. Geng et al., Opt. Lett., 36, 12, 2293–2295 (2011).
8. Q. Wang et al., Opt. Lett., 34, 23, 3616–3618 (2009).
Shibin Jiang is president and CEO of AdValue Photonics, 3708 E. Columbia St., Suite 100, Tucson, AZ 85714; e-mail: [email protected]; www.advaluephotonics.com.