PHOTONIC FRONTIERS: OPTICAL CLOCKS: Optical clocks set the pace in accurate timekeeping
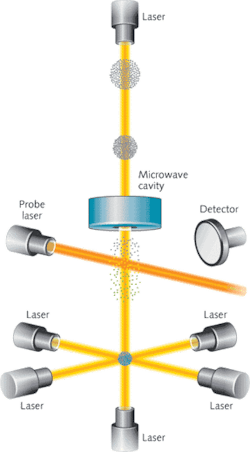
Atomic clocks based on a microwave transition of cesium have been the gold standard of timekeeping for decades. In 1967, metrologists formally defined the second as equal to 9,192,631,770 oscillations of a hyperfine transition of ground-state cesium-133. Since then researchers have made steady improvements in atomic-clock accuracy. The first cesium clock in 1955 was accurate to one part in 1010. Today, the primary time standard is an advanced cesium clock at the National Institute of Standards and Technology (NIST; Boulder, CO) called NIST-F1 (see Fig. 1). Lasers cool and confine a fountain of cesium atoms, limiting the clock’s fractional frequency uncertainty to a remarkable 4 × 10-16, corresponding to a drift of one second in more than 60 million years.1
But cesium clocks are coming up against fundamental limits. Their 9.2 GHz microwave frequency was high 50 years ago, but optical frequencies are 100,000 times higher so they can slice time into much smaller intervals. “It’s like adding five digits to your stop watch,” says Chris Oates, an optical-clock researcher at NIST in Boulder. Optical clocks are also about 100 times more stable than typical cesium clocks, which is a big advantage in achieving precise timing. Time averaging improves atomic-clock precision by smoothing out instabilities, but the precision improves only as the square root of the measurement time—so the more stable optical clock needs only a few seconds to reach 10-15 precision, compared to many hours for a microwave atomic clock. That’s a big plus for practical measurements.
Building blocks of optical clocks
Although the theoretical advantages of optical clocks have long been obvious, realizing that potential has required major innovations in all three fundamental building blocks of optical clocks: an atomic species with a narrow transition in the visible or ultraviolet, a laser with sub-hertz linewidth that can be tuned to match the atomic resonance, and a counter capable of measuring laser frequencies in the optical range.
Achieving high accuracy requires tools to limit the movement of the atoms being studied and holding them in place long enough for the measurement system to achieve high resolution. The development of laser cooling and laser trapping techniques succeeded in slowing and trapping atoms, greatly reducing Doppler shifts and extending interaction times. Advances in laser stabilization provided the required light source.
The final step was development of laser femtosecond frequency combs, which can directly measure the absolute optical frequency by dividing the frequency down to the microwave range. With these tools in hand, developers began demonstrating impressive performance in optical clocks, which in theory might reach accuracy of 10-17 to 10-18.Types of optical clocks
One type of optical clock is based on trapping and isolating a single atom and laser-cooling it to about one millikelvin. This slows the atom’s motion to near zero and confines it in a volume just a few tens of nanometers across—an order of magnitude shorter than optical wavelengths—which allows long interaction times with the excitation laser beam, as needed for accurate measurement.
Jim Bergquist and colleagues at NIST in 2000 demonstrated the first such optical clock, by electromagnetically confining mercury-199 ions between a pair of electrodes. The mercury ion oscillated on a transition near 282 nm with linewidth of only 7 Hz. After a series of refinements, the group showed its mercury clock could exceed the accuracy of the best cesium clocks (see Fig. 2).2 The precise control possible with a single atom promises very high accuracy, but the use of a single atom at a time can limit the signal-to-noise ratio and measurement precision. Other ionic optical clocks being studied include a strontium-88 line at 674 nm and ytterbium-171 ions at 435 nm. Both have demonstrated linewidths within a factor of 10 of the mercury line.A closely related clock type is the “quantum logic” optical clock developed at NIST, in which two different ions—aluminum-27 and beryllium-9—are held 4 µm apart in an electromagnetic trap and laser-cooled. The pump laser can be tuned to resonantly excite the aluminum ion to a higher state, but it’s hard to detect the aluminum state directly. Instead, the aluminum atom is coupled to the beryllium atom using a “quantum logic” operation that makes the beryllium atom emit easily detected light when the aluminum atom has been excited.
Last year the NIST group showed it could hold the frequency ratio of the quantum-logic and mercury clocks constant with fractional uncertainty limited to an impressive 5.2 × 10 -17. 3
An alternative approach is trapping ensembles of neutral atoms, which has been demonstrated for species including calcium, ytterbium, and a 698 nm line of strontium-87. Although using many atoms improves signal-to-noise ratio and measurement precision, many atoms are harder to control than one, and interactions among atoms can shift frequency and degrade system accuracy. To reduce these interactions, developers can confine individual atoms in separate traps in an optical lattice, an array of subwavelength optical potential wells created by the interference of two counterpropagating laser beams (see Fig. 3). The resonant laser and the cooling lasers illuminate the area of the optical lattice. By trapping thousands of atoms in individual potential wells where they are isolated from their neighbors, the optical array increases signal-to-noise ratio, offering better measurement precision.
The first optical-lattice clock, using a 698 nm line of strontium-87, measured a frequency of 429,228,004,229,952 ±15 Hz at the National Metrology Institute of Japan (Tsukuba, Japan).4 Further experiments at LNE-SYRTE, the French National Institute of Metrology (Paris) and at JILA at the University of Colorado (Boulder) refined those results. Last year, a team from JILA and NIST reduced the fractional uncertainty of the strontium-lattice clock to 1 × 10-16.5 The team established that value by all-optical comparison between the JILA strontium-lattice clock and a calcium-lattice clock at NIST through a 4 km optical-fiber link.
Applications of optical clocks
So far, optical clocks are research projects sitting in major research labs, including NIST, JILA, LNE-SYRTE, NMI-Japan, the U.K. National Physical Laboratory (Teddington, England), and the German Federal Physical-Technical Institute (Physikalisch-Technische Bundesanstalt; Braunschweig, Germany). Dedicated to fundamental research, those laboratories have already begun using optical clocks to push the state of the art in measurements.
One example is testing whether fundamental constants might vary over time. Astrophysicists must look out to the edge of the universe to study distant galaxies to seek evidence of such variations. But optical clocks can detect extremely small changes that might be continuing today. Each type of optical clock depends on fundamental constants in different ways, so comparing the time recorded by two different clocks can set an upper limit on the variation of these constants with time. Last year, measurements comparing NIST’s mercury clock with its aluminum “quantum logic” clock showed that over the course of a year the fine structure constant varied by only (-1.6 ±2.3) × 10-17—essentially zero within the range of measurement error.3 That’s significant news for fundamental physics.
“The best optical clocks are a factor of 10 better [more accurate] than cesium,” says Oates, but they’re not yet ready to replace the microwave cesium clock as the fundamental standard for measuring time. Cesium clocks have been compared for decades, so the long-term stability vital for fundamental standards has been well established. Optical clocks have performed well so far, but they’re newcomers. The world has only one mercury-ion clock and one aluminum-ion clock, and three strontium clocks. Moreover, Oates says, today’s cesium-fountain clock standards “give performance that very few users outside of national laboratories need right now.” Further development requires building more optical clocks, comparing their performance, and extending the distance over which optical clocks can be compared. In short, it’s going to take time to satisfy the requirements of international standards groups.
Outlook
The prospects for practical applications may be brighter. Developers of precision navigation systems in space or over long distances want clocks with femtosecond jitter to pinpoint locations better than today’s Global Positioning System. Optical clocks can achieve that goal in seconds because of their high frequencies; cesium takes much longer.
Commercialization will be a challenge. Today’s optical clocks are complex systems that require laboratory fine-tuning and adjustment. Developers want equipment that’s simple and rugged. But progress is being made, including the development of fiber lasers that can replace Ti:sapphire lasers for generating femtosecond frequency combs. Oates thinks the first optical clocks to reach the commercial world are likely to be used to generate a stable microwave signal for timing with lower noise than is possible from conventional microwave systems. And optical clocks are sure to open new possibilities.
REFERENCES
- S.A. Diddams et al., Science 306, p. 1318 (Nov. 19, 2004).
- W.H. Oskay et al, Phys. Rev. Lett. 97, p. 020801 (July 14. 2006).
- T. Rosenband et al., Science 319, p. 1808 (March 28, 2008).
- M. Takamoto et al., Nature 435, p. 321 (May 19, 2005) doi:10.1038/nature03541.
- A.D. Ludlow et al., Science 319, p. 1805 (2008); DOI: 10.1126/science.1153341.
Tell us what you think about this article. Send an e-mail to [email protected].
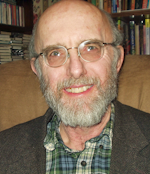
Jeff Hecht | Contributing Editor
Jeff Hecht is a regular contributing editor to Laser Focus World and has been covering the laser industry for 35 years. A prolific book author, Jeff's published works include “Understanding Fiber Optics,” “Understanding Lasers,” “The Laser Guidebook,” and “Beam Weapons: The Next Arms Race.” He also has written books on the histories of lasers and fiber optics, including “City of Light: The Story of Fiber Optics,” and “Beam: The Race to Make the Laser.” Find out more at jeffhecht.com.