QUANTUM TUNNELING: Photoelectron movies will feature chemical reactions
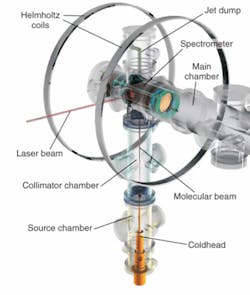
About a decade ago, Paul Corkum imagined a laser beam tearing an electron from a molecule and then driving the same electron back to the molecule to take its own picture. The idea seemed straightforward at the time. “The electron could hardly miss the molecule,” he said.
Ten years and a few details later, Corkum and colleagues at the National Research Council of Canada (NRC; Ottawa, Ontario, Canada), Goethe University (Frankfurt, Germany), and the National Institute for Scientific Research (Varennes, Quebec, Canada) have come up with a combination of laser-induced electron diffraction (LIED) and tunneling spectroscopy that might be thought of as a molecular camera, and, taking it even further, they’ve opened a route to a molecular movie camera—capable of shooting images at femtosecond frame rates—for real-time three-dimensional viewing of photochemical reactions in small molecules. Meanwhile Corkum’s imagination has already begun to extend the technique into the much larger and more complex world of biological molecules and reactions.
The molecular camera functions in two ways. First, in a manner analogous to the quantum tunneling of a scanning tunneling microscope (STM), electrons tunnel through vacuum from a metallic or semiconducting surface to the conducting tip of the scanning probe, yielding current variations in the probe that are then compiled into images. Unlike an STM, however, the molecular camera does not scan surfaces. Laser pulses extract electrons from a parent molecule and accelerate the electrons toward a detector. Second, the pulse oscillation also returns about half of the extracted electrons to the parent molecule, where they collide and create diffraction patterns.
The diffraction patterns generate image data for the nuclear structure of the parent molecule, and the momentum distribution of initially extracted electrons provides image data for the highest occupied molecular orbital. So the photoelectrons in the molecular camera simultaneously provide detailed information about both the orbiting electrons and the atomic nuclei of a molecule. Corkum wasn’t thinking of an STM, while developing the technique. The analogy occurred to him after the work was completed, and offers a useful point of reference for describing how the technique works.
“We can’t apply a static voltage as one would with an STM, but extracting electrons with an infrared-laser field is well approximated by tunneling,” he said. “In other words, we have the same physical process that you have taking place on the probe tip as it sits on the molecule. Also we can’t scan the electric field across the molecule, but we can rotate the molecule in angular increments around the nuclear axis. And that’s quite a close analog, if you think about it, to scanning the STM probe tip from the atom center to slightly off center and then a bit further, to the next atom. So we’re using the same physical technique, but applying it differently.”
Measuring the momentum distribution
A huge advantage of the laser method is that extracted electrons don’t disappear into the probe tip as they do in STM. “A good percentage of the electrons fly directly to the detector, where we can see all their characteristics,” Corkum said. “So we don’t only measure current, we measure the full momentum distribution. You obtain much more information.” The LIED methodology is not likely to compete with or replace the STM probe, however, which is better adapted for scanning material surfaces.
The initial experimental apparatus for the molecular camera was illuminated by an 800 nm laser source providing 5 μJ pulses at a 30 kHz repetition rate, and the source beam was split to provide both pump and probe signals. A 60 fs pump pulse was used to align oxygen and nitrogen molecules in a gas mixture, and a 40 fs probe signal extracted electrons from the gas molecules. The detector, a cold target recoil ion momentum spectroscopy (COLTRIMS) system, precisely measured three-dimensional electron momentum vectors (see figure). The precision of the initial experimental system was not necessary to achieve the initial goal of having an electron “take a picture of itself,” however.
“We have now done experiments with a different electron detector technology at 100 Hz,” Corkum said. “It could be done at a kilohertz. It could be done with a garden-variety femtosecond laser.”
The 40 fs pulse width in their experiments was not critical because they were not looking at molecular dynamics and not trying to make movies. But in principle, it’s the same technology that enables attosecond pulse generation and that would enable real-time observation of chemical and biochemical reactions. The researchers have also run the experiment using detection systems that only provided two-dimensional information, and achieved similar results. “You notice a difference due to different technology,” Corkum said. “But you do not notice a difference in the experimental results.”
Corkum described the group’s work so far as a proof-of-principle experiment in which the excitement arises from the demonstrated ability to simultaneously measure electronic and nuclear structure. “What happens during a photochemical reaction? Can we see the orbital, just slightly after we excite the molecule? Can we watch the chemical reaction as it occurs and see how the orbital changes, how the atoms move? We can take femtosecond snapshots of these processes, assemble them sequentially in frames like a movie that we watch on TV,” he said. “I think that’s where the excitement lies.”
REFERENCE
1. M. Meckel et al., Science 320, 1478 (June 13, 2008).
Hassaun A. Jones-Bey | Senior Editor and Freelance Writer
Hassaun A. Jones-Bey was a senior editor and then freelance writer for Laser Focus World.