Optogenetics/Brain Research: Optical developments take optogenetics deeper, less invasively
As neuroscientists progress in their application of optogenetics, they want to take it further. This is evidenced by an explosion in the development of light-sensitive opsins that allow researchers to target neurons with amazing specificity. The result has been an explosion in understanding the relationships between neural dynamics and behavior. But the old problem of light scattering is a hurdle in the path of brain research. At this point, optogenetics’ dramatic impact on neuroscience is being tenuated by the invasive use of optical fiber to reach beyond the brain’s superficial layer. Thank goodness for the ingenuity of engineers, as new and refined optical tools are increasing depth and reducing invasiveness simultaneously.
High-performance, low-profile implants
One avenue that engineers have been pursuing is the development of implantable optical neural interfaces.1 This year, Komal Kampasi, a University of Michigan (U of M; Ann Arbor, MI) engineering postdoc now working at Lawrence Livermore National Laboratory (LLNL), was the first author on a paper describing work by researchers at U of M and New York University (NYU) to develop an implant less invasive than optical fiber—and capable not only of controlling electrical activity deep in the brains of awake mice, but also of activating multiple opsins simultaneously with multicolored light.2
The National Institutes of Health (NIH; Bethesda, MD) funded the work under the White House BRAIN Initiative, targeting development of technology to better understand dense and deep brain regions. Kampasi’s team, headed by U of M professor Euisik Yoon, developed the multicolor neural optoelectrode, and the NYU team applied it to study the hippocampus. The design integrates micro-lasers with waveguides in a compact, scalable platform. The device achieves the optical, thermal, and electrical characteristics that have been a barrier in previous designs of fiberless optoelectrode implants.
Most optogenetics research has involved manipulating one type of neuron at a time using a single color of light, but the multicolor, fiberless device can control two or more neuron populations that are spatially integrated. The work represents an important advance because it gives neuroscientists multicolor optogenetic control over different neuron types “at a local circuit level while simultaneously allowing them to record high-quality, low-noise electrical data from those cells,” Kampasi explains. Thus, the technology promises to facilitate understanding of complex neural circuits’ organization and function.The high-density optoelectrode design incorporates four neural shanks and injection laser diode (ILD) light sources of 405 and 635 nm (see Fig. 1). GRIN lenses are used to couple the compact ILDs to monolithic dielectric optical mixer waveguides. Two ILDs for each of the four shanks enables high-quality recording during bench testing with a dramatic reduction in stimulation artifacts (under 100 μV) vs. the team’s previous design. Measuring 70 μm wide and 22 μm thick each, the four shanks were designed to minimize tissue damage. A 5 × 5 mm “jig” holds the eight ILD-GRIN pairs (a die-bonding tool enabled alignment of the ILDs and GRINs with sub-micrometer accuracy). Each of the shanks’ probes is integrated with eight recording sites as well as a two-color optical mixer with a 7 × 30 μm waveguide tip (see image at top of article).
Device application and development
At NYU, Gyorgy Buzsaki leads a team of neuroscientists with a track record of research on the hippocampus—one of those deep brain regions targeted by the BRAIN Initiative funding. Eager to understand how memories are formed and erased, Buzsaki’s team saw the device as a promising tool for studying the interaction of different hippocampal cell types and implanted it into the brains of awake mice—specifically to the section of the hippocampus called CA1. The researchers targeted two cell types two different opsins, and the researchers were able to excite both types of cells with sub-milliwatt illumination.
The team’s results demonstrate that the device enables neural circuit interrogation requiring parametric control of two neuron types in awake mammals. “We wanted to know if we could precisely control the spiking activity of densely intermingled neuron types in mice hippocampus,” Kampasi says. “We were ecstatic to see that we can.”
This ability to simultaneously and independently manipulate various categories of neurons at specific locations is key for understanding the relational dynamics of these different cell types. And thus, it enables new opportunities in neuroscience.
Those opportunities will be enhanced by further development of the technology. “With an ever-growing photonics industry, as bare laser chips become available in more wavelengths in [the] near future, our technology can be readily extended to conduct multi-opsin experiments,” the researchers wrote. One goal is to combine the technology with thin-film neural probes developed at LLNL, and another possibility is wireless circuit control.
Deep, flexible, and wireless
Both wireless operation and flexible probes are directions being pursued by other researchers, of course. In late 2017, an international group led by the University of Illinois at Urbana-Champaign (Champaign, IL) reported a flexible, wireless, injectable fluorescence photometer on a thin, needle-shaped polymer substrate designed for minimally invasive implantation deep into the brain.3 The device features an inorganic LED measuring 270 × 220 × 50 μm, a 100 × 100 × 5 μm photodetector, and a maximum thickness of ∼150 μm on the injectable part of the probe.
The researchers designed the device to measure calcium transient activity in any region of the brain that expresses a dynamic fluorescent protein (e.g., genetically encoded Ca2+ indicator), and in their tests applied it to the deeply seated basolateral amygdala (BLA) brain structure. Their results demonstrate the setup’s high-fidelity recording capabilities, which turns in measurement performance matching or exceeding that of the traditional fiber-based systems that restrict animal movement.
Deeply positioned nanoparticles convert light
Researchers at the RIKEN Brain Science Institute in Japan have developed another approach for delivering light deep into the brain: They harness upconversion nanoparticles (UCNPs) to serve as a handoff point in a relay of two light wavelengths, one capable of deep tissue penetration and the other capable of photoactivation.4 They apply tissue-penetrating near-infrared (near-IR) laser light transcranially to mice with UCNPs injected into areas of the brain not typically reachable except by invasive probes. The lanthanide-doped UCNPs convert the near-IR energy to wavelength-specific visible light capable of triggering nearby light-sensitive opsins.
Group leader Thomas McHugh and his colleagues developed the UCNPs by synthesizing NaYF4 nanocrystals, which they co-doped using either a combination of Yb3+ and Tm3+ (to provide blue emission needed for activating channelrhodopsin-2 [ChR2]; see Fig. 2), or a mix of Yb3+ and Er3+ (to produce the green light needed for absorption by halorhodopsin [NpHR]). They grew a NaYF4 shell over this core to make the particles optically inert. A final coating of silica (SiO2) or polyacrylic acid (PAA) makes the particles biocompatible and adds long-term viability.Testing their approach in mice, the researchers injected UCNPs into the brain, and transcranially applied near-IR laser pulses, which can be tuned for targeting of specific brain regions. The UCNPs absorbed the near-IR light and in turn activated and silenced labeled neurons, prompting dopamine release in the ventral tegmental area (VTA) ~4.2 mm beneath the surface. This produced brain oscillations by activating the medial septum’s inhibitory neurons, quashing seizure by inhibiting excitatory cells in the hippocampus, generating memory recall.
Using a 980 nm continuous-wave (CW) laser diode at 2.0 W, they produced a conversion yield that compares favorably with optical fiber for opsin activation. The researchers observed no cell damage or temperature increases, despite the fact that such light bursts concentrate energy in small areas.
The UCNPs are compatible with a variety of light-activated channels commonly used for optogenetics, and thus promise utility for neural activation or inhibition in many deep brain structures. “Nanoparticles effectively extend the reach of our lasers, enabling the ‘remote’ delivery of light and potentially leading to noninvasive therapies,” McHugh says. As part of their continued studies, the scientists are studying the particles’ chronic interaction with brain tissue.
These are just a few examples of work on the optical side of optogenetics that meet the increasingly refined needs of neuroscientists. As the optics advance, so will the research—toward the ultimate goal of true understanding of the brain.
REFERENCES
1. B. Fan and W. Li, Lab Chip, 19, 3838–3855 (2015).
2. K. Kampasi et al., Microsyst. Nanoeng., 4, 10 (2018).
3. L. Lu et al., Proc. Nat. Acad. Sci. (2017); doi:10.1073/pnas.1718721115.
4. S. Chen et al., Science, 359, 679–684 (2018).
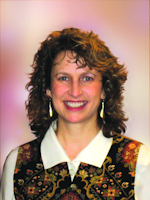
Barbara Gefvert | Editor-in-Chief, BioOptics World (2008-2020)
Barbara G. Gefvert has been a science and technology editor and writer since 1987, and served as editor in chief on multiple publications, including Sensors magazine for nearly a decade.