PHOTONICS APPLIED: BIOPHOTONICS: Optogenetics—Turning light bulbs on in the brain
When someone has an “aha!” moment, it’s as if a light bulb turns on in the brain. Even as chemists, biologists, and neuroscientists work to unravel this mysterious process of thought and revelation, researchers in the field of optogenetics are taking a more direct approach by artificially turning on those light bulbs—in a manner of speaking—to try and understand how the brain controls the physical body. A form of low-level light therapy (LLLT) or phototherapy less than a decade old, optogenetics is defined by Wikipedia as an emerging field combining optical and genetic techniques to probe neural circuits within intact animals at the high speeds (millisecond-timescale) needed to understand brain information processing. Here, the term “genetic” refers to the use of genetic methods to create and introduce light-sensitive proteins called rhodopsins into biological tissues.
According to the Massachusetts Institute of Technology (MIT; Cambridge, MA) Technology Review, some 500 laboratories worldwide are applying optogenetics to animal models.1 Early studies using light to control the movement of small nematode worms and fruit flies through neural stimulation have since been extended to mice and even primates, where optogenetics can be used to understand the spatial capabilities related to animal motion, as well as for wireless neural implants that are activated by miniature light-emitting diodes (LEDs). Optogenetics promises to restore vision to the blind, rescue nerve functions in patients with spinal-cord injuries, and to control the fear, motivation, and reward centers of the brain for possible addiction treatments. But just how does optogenetics differ from other methods of neural stimulation and how far from “commercialization” are its potential treatments?
Specificity
The algae protein channelrhodopsin can be introduced into neurons and activated with green light to turn them “on,” while halorhodopsin can be introduced and activated with yellow light to turn these neurons “off” at the frequency at which neurons operate.
“Unlike electrical stimulation, introducing a genetically encoded light responder such as channelrhodopsin allows neuroscientists to ‘choose’ to excite a subset of neurons within the region of stimulation,” says John Y. Lin, a postdoctoral research fellow with professor Roger Tsien at the University of California–San Diego (UCSD). “Because the limitation of the optical approach is the attenuation of light in mammalian tissues, I’ve been working to modify channelrhodopsins to improve their properties and move beyond 600 nm light for stimulation; this would reduce the light absorption by blood and tissue, allowing stimulation directly through the skull or skin without the need to insert fiber-optic probes,” he adds.2 Recently, David Tank and colleagues from Princeton University (Princeton, NJ) showed that two-photon stimulation of channelrhodopsin was possible at 920 nm infrared wavelengths.3
Besides wavelength modifications, Lin points out that improvements are needed in the production of virus-carrying channelrhodopsin for the introduction of these proteins into tissue: “Most physiology labs are not equipped to make the virus and viral production is typically labor intensive and costly. Purchasing an off-the-shelf stock of virus at a low price would be beneficial for optogenetics researchers.” Some nonprofit groups such as Addgene (Cambridge, MA) are distributing DNA and viruses that encode for light-gated proteins.
The specificity of optogenetic devices also allows them to work in concert with such techniques as blood-oxygenation-level dependent (BOLD) functional magnetic resonance imaging (fMRI), a form of fMRI that relies on intrinsic changes in hemoglobin oxygenation. “Recent work by Lee and colleagues showed that optogenetic stimulation of excitatory neurons triggers a positive BOLD response that can be monitored using fMRI,” says Helen Palmer, postdoc at the Norwegian University of Science and Technology (Trondheim, Norway).4, 5 “The findings confirmed that it’s possible to study a genetically defined neuron population of a particular brain region using so-called ‘optrodes’—optical fibers fused to recording electrodes that can simultaneously deliver the desired light wavelength to an exposed cranium while recording neural signals (see Fig. 1). Because our group has several projects using fMRI, including a large-scale project with traumatic brain injury survivors, it is important to validate the BOLD technique from a physiological standpoint in health and disease.” Palmer concludes, “Lee’s work gives physiologists and neuroscientists [who are skeptical of fMRI] empirical evidence to support the fact that the brain activation we ‘map’ in humans with fMRI is real!”
Brain implants for trauma recovery
Four universities, including Stanford University (Stanford, CA), Brown University (Providence, RI), the University of California–San Francisco (UCSF), and University College London, are the recipients of a Defense Advanced Research Projects Agency (DARPA) award that seeks to develop implantable therapies for brain trauma through optogenetics research.6 “By turning off certain parts of the brain to simulate a traumatic injury, we can study how the brain attempts to rewire itself and perhaps use this information to create prosthetic computer chips that could mimic the brain’s normal two-way communication to restore function,” says Krishna Shenoy, associate professor of electrical engineering at Stanford University and principal investigator of the REPAIR (Reorganization and Plasticity to Accelerate Injury Recovery) project, for which DARPA is providing $14.9 million for two years with an option to increase the project’s scope to $28.8 million and four years.
Primate optogenetics
In 2009, it was demonstrated that light applied to the frontal cortex of fully awake macaque monkeys that had been injected with lentivirus carrying the gene for channelrhodopsin-2 was expressed predominantly in excitatory pyramidal neurons.7 In layman’s terms, the research shows that particular neurons can be targeted, activated, and analyzed to understand brain/body function in a well-understood, well-studied, and trainable primate—and ultimately, in a human. Combined in vivo optical stimulation and electrophysiological recordings showed that the activity of neurons in the illuminated area could be influenced with millisecond temporal precision. Furthermore, the researchers found that the activity level of many neurons was suppressed following light exposure and concluded that this was due to the activation of inhibitory interneurons activated by the pyramidal neurons. It is precisely these types of neural interactions that optogenetics can highlight for researchers.
“Recent work has shown that rhodopsins do not illicit an immune response or lead to cell death in primates—an extremely critical finding that paves the way for future optogenetic study using these light-activated proteins,” says Ed S. Boyden, assistant professor of the MIT Media Lab and leader of the Synthetic Neurobiology Group at MIT who contributed to this primate research and also worked with Karl Deisseroth from Stanford University—who coined the term “optogenetics” in 2006—on early rhodopsin research. “We see our research lab as a core-technology provider to assist others with optogenetic research. To date, our achaerhodopsins, which overcome the fundamental limitations of nerve-quieting halorhodopsins such as weak signal and rundown over time, have been provided to around 250 research groups.”
Boyden continues, “Numerous research groups are now visiting MIT and collaborating to understand and access our newest tool—a multiwaveguide probe that can deliver specific wavelengths of light to multiple locations within tissue.”8 The 1.45 cm long probe is microfabricated in the form of a 360 μm wide array of 12 parallel silicon oxynitride (SiON) multimode waveguides coated with aluminum. The waveguide array sends light from a set of sources at the input end down each waveguide to an aluminum corner mirror that efficiently deflects light away from the probe axis (see Fig. 2).Optical pacemakers
Battery-operated electrical pacemakers that control the rhythmic operations of heart muscle are well understood. But a new optogenetic method is gaining traction not only as a less invasive research tool, but also as a potential light-based pacemaker that could outperform the conventional electrical alternative. “The new method allows us to silence or activate muscle and nervous tissues at will and with great temporal precision,” says Aristides Arrenberg from the neuroscience program at UCSF (see Fig. 3).9 “We can’t say at this point whether optical pacemakers will find a clinical application in the future. However, our recent results show the potential this new method could have if safe, therapeutic gene transfer becomes possible.”Researchers at the University of Bonn (Bonn, Germany) are also applying optogenetics to cardiac research.10 In their work, pulsed 475 nm blue light induces cellular contractions in channelrhodopsin-expressing cardiomyocytes both in vitro in a laboratory and in vivo within mice without side effects. By confined illumination of the ventricular and atrial regions of the beating heart of a mouse, the researchers were able to induce ventricular and supraventricular pacing, respectively. “Most importantly, we can now continuously activate cardiac tissue by constant illumination and therefore induce arrhythmias,” says Philipp Sasse, MD, at the University of Bonn. “Continuous activation of cardiac tissue is something completely new that cannot be done with electrical stimulation,” he adds.
No more blind mice
Eos Neuroscience (San Francisco, CA) is working in close collaboration with MIT Media Lab and other optogenetics research facilities to apply optogenetic innovations to intractable neurologic diseases in humans. Its first therapy, demonstrated in mice as a proof-of-concept experiment in 2008, aims to restore functional sight to patients blind from photoreceptor diseases such as retinitis pigmentosa (RP) and age-related macular degeneration (AMD). The Eos therapy enables the expression of an AAV-virus-delivered light-activated protein in retinal bipolar cells—a specific sub-population of neurons in the retina that receives input from photoreceptors. Even though the natural photoreceptors are gone, this therapy would allow the eye to again be activated by light. Eos Neuroscience co-founder and CEO Ben Matteo predicts that this technology could be in human clinical trials in two years.
REFERENCES
1. www.technologyreview.com/biomedicine/24644/
2. J.Y. Lin, Experimental Physiol., 96, 1, 19-25 (January 2010).
3. J.P. Rickgauer and David W. Tank, PNAS, 106, 35, 15025-15030 (Sept. 1, 2009).
4. J. Hyung Lee et al., Nature, 465, 788-792 (June 10, 2010).
5. H.S. Palmer, J. Neurophysiol., 104, 1838-1840 (2010).
6. http://news.stanford.edu/news/2010/may/repair-brain-injury-050410.html
7. X. Han et al., Neuron, 2, 191-198 (2009).
8. A.N. Zorzos et al., Opt. Lett., 35, 24, 4133-4135 (December 2010).
9. A.B. Arrenberg et al., Science, 330, 971-974 (November 2010).
10. T. Bruegmann et al., Nature Methods, 7, 897-900 (2010).
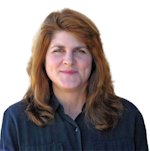
Gail Overton | Senior Editor (2004-2020)
Gail has more than 30 years of engineering, marketing, product management, and editorial experience in the photonics and optical communications industry. Before joining the staff at Laser Focus World in 2004, she held many product management and product marketing roles in the fiber-optics industry, most notably at Hughes (El Segundo, CA), GTE Labs (Waltham, MA), Corning (Corning, NY), Photon Kinetics (Beaverton, OR), and Newport Corporation (Irvine, CA). During her marketing career, Gail published articles in WDM Solutions and Sensors magazine and traveled internationally to conduct product and sales training. Gail received her BS degree in physics, with an emphasis in optics, from San Diego State University in San Diego, CA in May 1986.