Fluorescence Imaging/Microscopy: Improving fluorescence signal in time-sensitive experiments
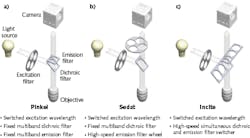
Multicolor fluorescence microscopy is foundational to a broad range of biological studies, thanks in large part to the staining specificity and resulting image contrast enabled by modern fluorescent labels. Despite this broad acceptance, though, selecting hardware for a particular experiment can be formidable. Cameras, light sources, and objectives are commonly discussed, while a less obvious, and often overlooked, aspect—optical filtering—affects scientific observation every bit as much.
So that you can effectively navigate the tradeoffs in performance and implementation complexity, let’s assess wavelength management options, compare images obtained using different configurations, and explore why some images look better or worse than others—using a familiar imaging target for quantitative comparisons so that you can relate the results to your own experience. Along the way, we’ll discuss recent technology advances and some challenging applications.
Image train and wavelength selection
Designers of fluorescence experiments can choose among several wavelength-filtering configurations for imaging multilabeled specimens. Criteria for selection include cost, performance, and implementation simplicity.
A traditional approach involves multiple filter cubes, each populated with a single-band filter set dedicated to an individual fluorophore and loaded in a manually actuated or motorized carousel. In terms of signal quality, this solution is exceptional, delivering excellent in-band throughput and out-of-band blocking. But the delay associated with switching cubes is sometimes unacceptable.
Fast image acquisition, which challenges efficient wavelength management, can be approached in several ways (see Fig. 1). The excitation light path, identical in the three examples, consists of a white light source coupled with a high-speed filter wheel (HSFW). Equivalent function (but with faster operation) can be achieved with electronically switched light-emitting diodes (LEDs) or acousto-optically switched lasers integrated in multiwavelength light combiners. The Pinkel configuration (see Fig. 1a) represents the simplest experimental setup with fixed multiband dichroic and emission filters. The Sedat arrangement (see Fig. 1b) also uses a fixed multiband dichroic filter—but emission light is adjustable, thanks to a HSFW that facilitates switching among single-band filters. Incite (see Fig. 1c), a recent technology advance from Finger Lakes Instrumentation (FLI; Binghamton, NY), exchanges single-band emission and dichroic filters—that are mounted on linear sliders—at speeds previously possible only with HSFWs.
Image quality tradeoffs
For applications that require high-throughput imaging, researchers have typically preferred Pinkel or Sedat configurations over the single-band filter sets used in relatively slower filter turrets. However, single-band filters offer distinct advantages over Pinkel and Sedat, as they maximize signal throughput and signal-to-noise ratio (SNR) while minimizing bleedthrough between colors.
A study comparing the approaches used a commercially available slide prepared with bovine pulmonary artery endothelial (BPAE) cells labeled using DAPI, Alexa 488 phalloidin, and MitoTracker Red CMXRos fluorescent stains. We imaged this slide using a variety of optical filter combinations, while keeping the illumination, camera, optics, and equipment settings consistent.
While the Pinkel configuration is the simplest to implement in hardware and can operate without any moving parts affecting the dichroic beamsplitter and emission filter, it can potentially introduce significant performance limitations. An example is the bleedthrough phenomenon, in which an image is contaminated with fluorescence signal from adjacent channels. This condition, also referred to as crosstalk, is caused by an overlap in the absorption and emission spectra of dyes in the specimen. Inherent in simultaneous transmission of several distinct wavelength bands by the multiband emission filter, it complicates image analysis and may lead to misinterpretation of results. The example presented in Fig. 2 illustrates how the DAPI excitation filter also excites the Alexa 488 and MitoTracker Red fluorophores (absorption corresponds to the green and red area plot segment under dashed lines in Fig. 2d between 350 and 405 nm), whose emission light passes through the multiband emission filter (see Fig. 2d, solid yellow line). The results are undesirable.Bleedthrough is virtually eliminated in the Sedat and Incite arrangements, which use single-band emission filters designed to transmit light associated with only one signal of interest (see Fig. 2d, solid red line). This experiment applied identical multiband dichroic beamsplitters in the Pinkel and Sedat configurations, whereas Incite incorporated a single-edge, longwave-pass dichroic optimized for DAPI.
The practical difference becomes apparent in a side-by-side comparison (see Fig. 3). Signal improvement arises from the relationship between the fluorophores’ absorption and emission spectra and the transmission spectra of the excitation and emission filters (see Fig. 4). Although the excitation filter bandwidths are wider with Incite than Sedat, that benefit is moot with narrow-wavelength light sources.
Emission band effects
The more substantial effect, and one that is applicable to any type of illumination, emerges from the significant broadening of the emission passband (solid blue line vs. solid red line in Fig. 4a and 4b). Specifically, the emission wavelength range of the all-single-band Incite configuration extends into the excitation bandwidth of the adjacent channel (area between the solid yellow line plots in Fig. 4). Signal from this emission band is lost in the Pinkel and Sedat configurations because the dichroic filter reflects this portion of the spectrum to facilitate excitation light delivery for the adjacent fluorescence channel. The improvement in signal quality represents a valuable addition to the system designer’s toolset, and to the knowledge base concerning imaging component and protocol tradeoffs and decisions.The difference in data obtained with a system using dedicated single-band dichroics (Incite) compared to a multiband one (Sedat) is equivalent to doubling or even quadrupling of camera quantum efficiency (QE), depending on experiment chemistry, with all other components and acquisition parameters being equal. In addition to resolving more signal at short exposures and enabling higher frame rates and temporal resolution in dynamic cellular process studies, the ability to switch dichroics at the speed of emission filters can reduce image acquisition by a factor of two or more in high-content screening applications. Such quick switching can also reduce photobleaching or phototoxicity of biological samples.
Microscope system integrators already maximize system-level throughput by using high-QE cameras and high-numerical-aperture (NA) objectives with excellent transmission characteristics. Incite provides another powerful tool for high-speed imaging. While the Incite arrangement represents a substantial and sought-after improvement in these systems, the simplicity of the practical implementation makes it suitable for many research microscopes by directly replacing an existing cube turret.
Hardware implementation
Critical performance characteristics of some microscope system components are easily quantifiable. For instance, it is not difficult to compare costs and benefits of two cameras, one with 82% QE and one that captures 95% of incoming light.
With other components, the situation is more complicated because of the number of factors and their interdependencies that affect the outcome of the experiment. Fluorescence filter performance—in terms of in-band transmission, out-of-band blocking, and steepness of the transition between transmission and blocking—is key to achieving best possible outcomes. However, the number of available choices is overwhelming, even if only best-in-class filters are considered.
When designing a fluorescence-based study, interactive online tools such as Semrock’s SearchLight (https://searchlight.semrock.com) are indispensable for navigating the complex landscape of parameters. They contain a vast database of existing fluorophores, filters, light sources, and detectors, and provide the ability to simulate the result of an experiment based on a selected combination of system components. Comparisons between a number of expected outcomes corresponding to different component combinations provide the necessary insight into selecting the right filters corresponding to different imaging configurations.
For time-sensitive observations, additional factors need to be considered, including the dynamics of the observation limiting the camera exposure, sensor readout time, detection signal limit, and filter exchange time. Table 1 provides a general overview of traditional wavelength management solutions, including single-band filter cube turrets and HSFWs, along with recent innovations such as FLI’s galvanometer-based emission optical path switcher and the Incite dichroic and emission filter switcher.
Table 2 summarizes the specifications for hardware options pertaining to the triple-stained sample used in our demonstration. Preferred qualities—such as improved signal intensity produced by single-band dichroics or short filter change time—are highlighted in green, while inferior performance—such as bleedthrough observed with multiband emission filters or long filter switching time—is highlighted in red. These parameters, combined with nominal camera exposure time, explain the quantitative differences observed in images obtained with the different configurations.
Figure 5a shows cumulative signal intensity as a function of nominal camera exposure. The intersection of the plots with a horizontal line allows for relative comparison of the exposure required to achieve a given signal level using different wavelength management components. For example, a system with the Incite switcher acquires the same level of Alexa 488 signal in 50 ms as a Sedat system acquires in 100 ms. Figure 5a also shows that a standard single-band filter cube turret produces higher signal intensities than a fast-filter-wheel Sedat solution at nominal exposures over 400 ms.Signal intensity normalized with respect to exposure time in Fig. 5b provides for a comparison of the hardware options at a given exposure time as an intersection of the plots with a vertical line. It shows that the Incite configuration produces highest intensity signal at all nominal exposures over 40 ms, a factor-of-two improvement over 23 ms-adjacent exchange Sedat at 40 ms, and more than threefold improvement over Sedat at exposures over 60 ms.
High speed, high content
Recent technology developments deliver considerable improvements in fluorescence signal quality, especially in high-speed and high-content systems. While traditional filter cube turrets perform well in experiments with relatively long exposures (over 400 ms), the dichroic and emission filter-switching Incite solution excels in experiments with short exposures (40–400 ms). In the space of very short exposures (<40 ms), best performance can be obtained using galvanometer-based emission path switchers or simultaneous-exposure-capable image splitters as alternatives to multicolor imaging solutions.
About the Author
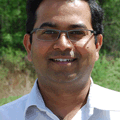
Prashant Prabhat, Ph.D.
Business Line Leader for Semrock Catalog
Prashant Prabhat, Ph.D. is Business Line Leader of Catalog Business at Semrock. Since 2008 he has been an Applications Scientist at Semrock. He earned his Ph.D. in Electrical Engineering from the University of Texas at Dallas and completed graduate research at Ward Lab at the University of Texas Southwestern Medical Center. It was there that he invented multifocal plane microscopy (MUM), a technique that allows for real-time visualization of fast cellular dynamics in 3D. MUM has enabled the discovery of novel trafficking pathways in cells, and formed the basis for 3D super-resolution techniques. At Semrock, he advises researchers on optical filter issues from a systems perspective, and helps develop the company’s product roadmap. As a new venture manager, he also has led the development of a computer controlled tunable filter system.
Jozef Sofka, Ph.D.
Senior R&D Engineer, Finger Lakes Instrumentation
Jozef Sofka, Ph.D., is a senior R&D engineer at Finger Lakes Instrumentation (Binghamton, NY).