High-performance near-infrared Raman for clinical application
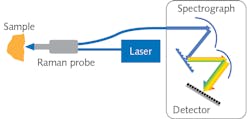
Raman spectroscopy has seen tremendous growth in biological and life science applications over the past two decades. As it measures inelastic scattering from a monochromatic light source incident onto a sample, Raman spectroscopy provides rich information on molecular structures, identities, and composition, and can be used for both qualitative and quantitative chemical analysis. This information, indicated by the wavelength difference (or relative Raman shift) and intensity of the scattering, results from interactions between photons and molecular vibrations.
This label-free, noninvasive technique has been widely used in research, and has more recently been pursued for its potential as an alternative or complementary clinical tool for patient care and disease diagnostics. This article will discuss the unique requirements for a medical Raman instrument, recent innovations in hardware development, and clinical cancer diagnostic applications enabled by these technologies.
Raman spectroscopic systems
Compared to other optical spectroscopy methods, Raman spectroscopy is considered a weak phenomenon with a relatively low cross-section (<1 × 10-8 ratio of Rayleigh vs. Raman scattering). High-sensitivity Raman spectrometers have been at the heart of successful research and application development. A Raman spectrometer comprises a laser source, spectrograph, and a detector (see Fig. 1). Although free-space coupling is used in many Raman systems, fiber-optic probes are widely used in medical diagnostics because of their flexibility and collection efficiency enabled by fiber bundling. Progress in hardware and software development—including high-throughput spectrographs, sensitive detectors with high quantum efficiency and low dark noise, novel sampling interfaces, and robust chemometrics tools—have made Raman spectroscopy an increasingly practical and powerful tool for complex biological cell- and tissue-related research.
Compared to conventional diagnostic methods used in medicine, Raman spectroscopy has potential to enable both in vivo and ex vivo diagnostics with high accuracy and high sample throughput at a much lower cost, with no sample preparation—capabilities that are either technically impossible or time- and cost-prohibitive using conventional methods (see Table 1). With the ability to monitor various diseases and key health indicators, Raman spectroscopy can provide real-time information for surgical guidance, drug effectiveness measurement, and point-of-care diagnostics when aided by an appropriate novel sampling interface.Designing a Raman spectrometer for a clinical environment requires consideration of the unique optical properties of biological tissues:
- Very high water content
- Highly fluorescent in the visible (VIS) region
- Highly scattering
- Strongly absorbent in the UV-VIS region
Excitation laser
Autofluorescence from biological specimens introduces severe interference to Raman spectral data and obscures analysis of results. However, longer-wavelength excitation lasers can effectively reduce or eliminate it. As longer wavelengths penetrate much deeper than UV-VIS light, Raman spectral data collected with near-infrared (near-IR) lasers have more biochemical information needed for accurate in vivo under-skin, tissue, and tumor analysis. Two optical windows in the near-IR spectral range are recommended for Raman spectroscopy (see Fig. 2). The first, and most commonly used, lasers for Raman excitation are 785 or 830 nm lasers, which overlay well within the first optical window. The second are 1064 nm lasers, which are used when autofluorescence is severe with shorter wavelengths. The choice of laser also relies heavily on the appropriate detector, which will be discussed in the next section.For in vivo measurements, the selection of laser power is determined by a few factors:
- Maximum permissible exposure
- Best signal intensity within the safety allowable power level
- Comfort level
- Local heating effect
For ex vivo measurements, a higher laser power may be used to improve the signal intensity.
Since biological tissues can have complex textures and can be highly scattering, both multi- and single-mode lasers may be used for a clinical Raman system. The latter are preferred for Raman microscopic measurements when high spatial resolution is required.
While near-IR light greatly reduces fluorescence interference, signal strength decreases with longer wavelength excitation because the spontaneous Raman scattering cross-section is proportional to 1/λ4, which makes the already ‘weak’ Raman signal even weaker in the near-IR. Also, water has a small Raman cross-section and human tissue is rich with water. This further reduces the Raman scattering from human issue. One can potentially increase laser power to improve Raman signal to certain level, but safety concerns and highly photon-sensitive tissues prohibit using a high-power laser. All these challenges point to a high-performance near-IR Raman spectrometer as the fundamental requirement for a successful clinical instrument.
Spectrograph
Dispersive grating-based spectrographs have several advantages over Fourier transform interferogram-based systems, and they are more broadly used as medical diagnostic tools due to simple optical design, lack of moving parts, and fast data collection with a focal-plane array detector.
Biological tissues are composed of mainly water with large molecules of proteins, lipids, and carbohydrates. Therefore, the Raman spectra from biological samples typically have broad peaks and features and do not need a long focal-length and high-resolution spectrograph.
On the other hand, a spectrograph with high throughput of light and low f/N is more desirable for high signal-collection efficiency. In addition, high-quality antireflective coating of the optics, aberration-corrected spectrograph design, and high efficiency gratings are critical to achieve the highest light throughput.As an example, Teledyne Princeton Instruments’ TPIR-785 Raman spectrometer incorporates a near-IR optimized lens spectrograph and thus easily enables a 40–60% light throughput improvement throughout the spectral range (see Fig. 3).
The TPIR-785’s spectrograph uses custom designed lens optics for aberration correction and provides imaging quality far superior to that of conventional Czerny-Turner spectrographs (see Fig. 4). Direct benefits of such high-quality imaging include:
- Multichannel or Raman imaging measurement with minimum crosstalk
- Stable high etendue and resolution across the entire spectral range and detector focal plane
- Works seamlessly with a Raman probe with a large fiber bundle and truly benefits from state-of-the-art large-format detectors
Detectors and probes
For high-performance spectrometers, charge-coupled device (CCD) detectors still provide the best quantum efficiency (QE) and lowest dark current with proper cooling in the UV-VIS region (see Fig. 5). However, the CCD QE starts to decrease at ~800 nm and has a cutoff at ~1100 nm, which is not quite efficient for near-IR Raman measurements. A high QE detector is desired for 785–1100 nm in order to effectively cover the fingerprint Raman spectral region (150–1500 cm-1). The TPIR-785 has the option to select a recently developed super-deep-depletion CCD camera (BLAZE HR) as its detector. The depletion region of epitaxial silicon is much thicker than the typical back-thinned CCD that drives QE in the near-IR region to 2–7X higher than conventional CCD detectors. The state-of-the-art camera also provides deep cooling to -95°C with air for lowest dark current. The high QE in near-IR region and low dark current makes BLAZE HR optimal for near-IR Raman. Additionally, the camera provides ultra-high readout speed through dual ports for fast spectral rate, which is critical for real-time diagnostics and Raman imaging.Cancer diagnostics and other applications
The noninvasive, simple, fast, and highly reproducible characteristics of its measurements make Raman spectroscopy a strong candidate for high-profile applications including cancer diagnostics, microbiology identification, and noninvasive blood glucose measurement.3,4
A large amount of research, covering nearly every aspect of medical diagnostics, has been dedicated to clinical Raman spectroscopic applications over the past decade. Pioneering studies using Raman spectroscopy for detection of cancerous tissue can be found as early as the 1990s.6,7 Since then, cancer diagnostics has become one of the most prolific research areas:4,2 The number of publications on Raman spectroscopy for cancer diagnostics has grown from less than 4000 in 2010 to more than 14,000 in 2019. Highlights in this field include:
More is needed, however, for Raman to fulfill its promise. It is interesting to reflect that the modernization of pharmaceutical manufacturing, through the adoption of optical spectroscopic process analysis tools, required a joint effort involving research communities, equipment vendors, pharmaceutical companies, and regulatory bodies for decades before the technologies finally became viable for the industry.
Among near-term challenges that will help Raman spectroscopy gain acceptance for clinical application are cost reduction; robust chemometrics/artificial intelligence/machine learning tools; size, weight, and power reduction; and regulatory acceptance.
REFERENCES
1. K. Lin et al., Theranostics, 7, 14, 3517–3526 (2017).
2. E. Cordero et al., J. Biomed. Opt., 23, 7, 1-23 (2018).
3. H. J. Butler et al., Nat. Protoc., 11, 664–687 (2016).
4. I. Pence et al., Chem. Soc. Rev., 45, 1958–1979 (2016).
5. A. N. Bashkatov, J. Phys. D: Appl. Phys., 38, 2543 (2005).
6. M. S. Feld et al., Advances in Fluorescence Sensing Technology II, 2388, 99–104 (1995).
7. A. Mahadevan-Jansen et al., International Conference of the IEEE Engineering in Medicine and Biology Society, 6, 2722–2728 (1997).
Peng Zou, Ph.D., is Product Manager at Teledyne Princeton Instruments, Trenton, NJ; e-mail: [email protected]; princetoninstruments.com.
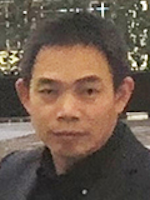
Peng Zou, Ph.D. | Product Manager
Peng Zou, Ph.D., is the Product Manager for the FERGIE Spectroscopy Systems at Teledyne Princeton Instruments.